the Creative Commons Attribution 4.0 License.
the Creative Commons Attribution 4.0 License.
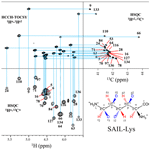
Conformational features and ionization states of Lys side chains in a protein studied using the stereo-array isotope labeling (SAIL) method
Mitsuhiro Takeda
Yohei Miyanoiri
Tsutomu Terauchi
Masatsune Kainosho
Although both the hydrophobic aliphatic chain and hydrophilic ζ-amino group of the Lys side chain presumably contribute to the structures and functions of proteins, the dual nature of the Lys residue has not been fully investigated using NMR spectroscopy, due to the lack of appropriate methods to acquire comprehensive information on its long consecutive methylene chain. We describe herein a robust strategy to address the current situation, using various isotope-aided NMR technologies. The feasibility of our approach is demonstrated for the Δ+PHS/V66K variant of staphylococcal nuclease (SNase), which contains 21 Lys residues, including the engineered Lys-66 with an unusually low pKa of ∼ 5.6. All of the NMR signals for the 21 Lys residues were sequentially and stereospecifically assigned using the stereo-array isotope-labeled Lys (SAIL-Lys), [U-13C,15N; β2,γ2,δ2,ε3-D4]-Lys. The complete set of assigned 1H, 13C, and 15N NMR signals for the Lys side-chain moieties affords useful structural information. For example, the set includes the characteristic chemical shifts for the 13Cδ, 13Cε, and 15Nζ signals for Lys-66, which has the deprotonated ζ-amino group, and the large upfield shifts for the 1H and 13C signals for the Lys-9, Lys-28, Lys-84, Lys-110, and Lys-133 side chains, which are indicative of nearby aromatic rings. The 13Cε and 15Nζ chemical shifts of the SNase variant selectively labeled with either [ε-13C;ε,ε-D2]-Lys or SAIL-Lys, dissolved in H2O and D2O, showed that the deuterium-induced shifts for Lys-66 were substantially different from those of the other 20 Lys residues. Namely, the deuterium-induced shifts of the 13Cε and 15Nζ signals depend on the ionization states of the ζ-amino group, i.e., −0.32 ppm for Δδ13Cε [NζD-NζH] vs. −0.21 ppm for Δδ13Cε [NζD2-NζH2] and −1.1 ppm for Δδ15Nζ[NζD-NζH] vs. −1.8 ppm for Δδ15Nζ[NζD2-NζH2]. Since the 1D 13C NMR spectrum of a protein selectively labeled with [ε-13C;ε,ε-D2]-Lys shows narrow (> 2 Hz) and well-dispersed 13C signals, the deuterium-induced shift difference of 0.11 ppm for the protonated and deprotonated ζ-amino groups, which corresponds to 16.5 Hz at a field strength of 14 T (150 MHz for 13C), could be accurately measured. Although the isotope shift difference itself may not be absolutely decisive to distinguish the ionization state of the ζ-amino group, the 13Cδ, 13Cε, and 15Nζ signals for a Lys residue with a deprotonated ζ-amino group are likely to exhibit distinctive chemical shifts as compared to the normal residues with protonated ζ-amino groups. Therefore, the isotope shifts would provide a useful auxiliary index for identifying Lys residues with deprotonated ζ-amino groups at physiological pH levels.
- Article
(7506 KB) - Full-text XML
- BibTeX
- EndNote
Detailed studies on the structures and dynamics of the Lys residues in a protein have been severely hampered by the difficulty in gathering comprehensive NMR information on their side-chain moieties. It is especially challenging to establish unambiguous stereospecific assignments for the prochiral protons in the four consecutive methylene chain, which is the longest aliphatic chain among the 20 common amino acids. Given the lack of generally applicable strategies to overcome this obstacle, only a few NMR studies have probed the structural aspects of stereospecifically assigned Lys residues. The ionization states of the Lys ζ-amino groups also provide important information, as they are often involved in specific intra- and/or intermolecular molecular recognition processes and thus play vital roles in protein functions. Therefore, the side-chain moieties of Lys residues contribute to maintaining the structure and biological functions of a protein by two elements: the hydrophobic methylene chain and the hydrophilic ζ-amino group. To investigate this dual nature of the Lys side chain, we have applied various isotope-aided NMR technologies, including the stereo-array isotope labeling (SAIL) method (Kainosho et al., 2006).
The Lys ζ-amino groups, which usually have pKa values around 10.5, are protonated (NH) at around neutral pH. However, certain proteins have Lys residues with deprotonated ζ-amino groups, even at neutral or acidic pH (Harris and Turner, 2002). In such cases, the pKa values of the Lys ζ-amino group are substantially lowered owing to its particular local environment. Since the Lys ζ-NH2 groups are endowed with significantly different physical chemical properties, as compared to the ζ-NH, they can perform specific functions, such as Schiff base formation through nucleophilic attacks on various substrates (Highbarger et al., 1996; Barbas et al., 1997). Although the ionization states of Lys ζ-amino groups in a protein have been inferred from X-ray crystallographic maps, they are subject to misinterpretation and may not always be identical to those in solution. NMR spectroscopy provides methods for determining the charge state of Lys side chains. For example, the NH and NH2 states of Lys residues in solution can be identified from cross-peak patterns in the 1H–15N correlation NMR spectra, if the hydrogen exchange rates are sufficiently slow, or from the values of 15Nζ and/or 1Hζ chemical shifts (Poon et al., 2006; Iwahara et al., 2007; Takayama et al., 2008). Under physiological conditions, however, the observations of 1H–15N cross peaks are often hampered due to the rapid hydrogen exchange rates of the Lys ζ-amino groups (Liepinsh et al., 1992; Liepinsh and Otting, 1996; Otting and Wüthrich, 1989; Otting et al., 1991; Segawa et al., 2008). The ionization states can also be identified by the pH titration profiles for the 13Cε and 15Nζ signals of individual Lys residues (Kesvatera et al., 1996; Damblon et al., 1996; Farmer and Venters, 1996; Poon et al., 2006; Gao et al., 2006; André et al., 2007). Unfortunately, long-term experiments such as pH titrations are hampered by the stability and solubility issues of a protein over the required pH range. Therefore, straightforward and robust alternative methods to identify Lys residues with distinct ionization states for the ζ-amino groups are highly desired.
We used a variant of staphylococcal nuclease, Δ+PHS/V66K SNase (denoted as the SNase variant hereafter), as the model protein (Stites et al., 1991). This variant was engineered to add the following three features to the wild-type SNase: (i) introduction of three stabilizing mutations, P117G, H124L, and S128A (PHS); (ii) deletion of amino acids 44–49 and introduction of two mutations, G50F and V51N (Δ); and (iii) substitution of Val66 with Lys (V66K). With these three modifications, the Δ+PHS/V66K SNase variant becomes thermally stable, even with the ζ-amino group of Lys-66 entrapped within the hydrophobic cavity originally occupied by the Val-66 side chain in the wild-type SNase. As a result, the ζ-amino group of Lys-66 in the SNase variant exhibits an unusually low pKa value of 5.7 (García-Moreno et al., 1997; Fitch et al., 2002).
Although the SNase variant contains 21 Lys residues (Fig. A1), including the engineered Lys-66, the 13C, 1H, and 15N NMR signals for the Lys side chains were unambiguously observed and assigned using the SNase variant selectively labeled with SAIL-Lys, i.e., L-[U-13C,15N; β2,γ2,δ2,ε3-D4]-Lys (Kainosho et al., 2006; Terauchi et al., 2011). In this article, we examine some of the structural features inferred from the comprehensive chemical shift data and the deuterium-induced isotope shifts on the 13Cε and 15Nζ of the Lys residues in the SNase variant and show that the side-chain NMR signals can serve as powerful probes to investigate the dual nature of a Lys side chain in a protein.
2.1 Sample preparation
The Δ+PHS/V66K SNase variants selectively labeled with either L-[U-13C,15N]-Lys, L-[U-13C,15N; β2,γ2,δ2,ε3-D4]-Lys (SAIL-Lys), or L-[ε-13C;ε,ε-D2]-Lys, which were synthesized in-house, were prepared using the E. coli BL21 (DE3) strain transformed with a pET3 vector (Novagen), encoding the Δ+PHS/V66K SNase gene fused with an N-terminal His-tag. The transformed E. coli cells were cultured at 37 ∘C in 500 mL of M9 medium, containing anhydrous Na2HPO4 (3.4 g L−1), anhydrous KH2PO4 (0.5 g L−1), NaCl (0.25 g L−1), D-glucose (5 g L−1), NH4Cl (0.5 g L−1), thiamine (0.5 mg L−1), FeCl3 (0.03 mM), MnCl2 (0.05 mM), CaCl2 (0.1 mM), and MgSO4 (1 mM), with 10 mg L−1 of the monohydrochloride salts of either [U-13C,15N]-Lys, SAIL-Lys, or [ε-13C;ε,ε-D2]-Lys. Each culture was maintained at 37 ∘C. An additional 20 mg L−1 of each isotope-labeled Lys was supplemented when the OD600 reached 0.5, and then protein expression was induced by adding isopropyl-β-D-thiogalactopyranoside (IPTG) to a final concentration of 0.4 mM. At 4–5 h after the induction, the cells were collected by centrifugation, and the SNase variant proteins were purified on a Ni-NTA column (Isom et al., 2008). The enrichment levels for Lys were ∼ 70 %, as measured using mass spectrometry. The purified proteins were dissolved in 20 mM sodium phosphate buffers containing 100 mM KCl (pH 8.0), together with a small amount of DSS as the internal chemical shift reference, prepared with either H2O, D2O, or H2O : D2O (1 : 1). The chemical shifts for 1H, 13C, and 15N were primarily referenced to the methyl proton signal of the internal DSS according to the IUPAC recommendation (Markley et al., 1998). However, we usually convert the δDSS (1H 13C) to δTSP (1H 13C) simply by adding 0.15 ppm, i.e., δDSS–δTSP = 0.15 ppm, for adjustment to the previous δTSP–(1H) chemical shifts reported for wt (wild-type) SNase (Torchia et al., 1989). On the other hand, all of the 15N chemical shifts are referenced to DSS, to facilitate the chemical shift comparison with the recent 15N data (Takayama et al., 2008). The chemical shift references are mentioned in the footnotes of the figures and tables.
2.2 NMR spectroscopy
The 600 MHz 2D 1H–13C constant-time heteronuclear single quantum coherence correlation (HSQC) spectra of the SNase variant, selectively labeled with either [U-13C,15N]-Lys or SAIL-Lys, were measured in D2O at 30 ∘C on a Bruker Avance spectrometer equipped with a TXI cryogenic probe. For the latter sample, additional deuterium decoupling was applied during the t1 period. The data sizes and spectral widths were 1024 (t1) × 2048 (t2) points and 12 000 Hz (ω1, 13C) × 8700 Hz (ω2, 1H), respectively. Each set of 32 scans per free induction decay was collected with a 1.5 s repetition time, using the 13C carrier frequency at 38 ppm. The 600 MHz 3D HCCH total correlation spectroscopy (TOCSY) spectrum was measured in D2O at 30 ∘C for the SNase variant labeled with SAIL-Lys (Clore et al., 1990; Cavanagh et al., 2007). The data size and spectral width were 1024 (t1) × 32 (t2) × 2048 (t3) points and 6000 Hz (ω1, 1H) Hz × 9100 Hz (ω2, 13C) × 9000 Hz (ω3, 1H), respectively. Each set of 16 scans/FID with a 1.5 s repetition time was collected, using the 13C carrier frequency at 40 ppm.
The Lys ζ-15N signals of the SAIL-Lys-labeled SNase variant dissolved in D2O at 30 ∘C were assigned using the HECENZ pulse sequence, utilizing the out-and-back magnetization transfer from 1Hε2 to 15Nζ via 13Cε. The correlations between the 1Hε2 and 15Nζ signals for most of the 21 Lys residues were firmly established by the pulse sequence, which was basically the same as the H2CN pulse sequence developed by André et al. (2007). The data size and the spectral width were 512 (t1) × 1024 (t2) points and 1200 Hz (ω1, 15N) Hz × 9600 Hz (ω2, 1H), respectively, and deuterium decoupling was applied during the t1 period. The carrier frequencies were 38 and 28 ppm for 13C and 15N, respectively, and 128 scans/FID with a 2 s repetition time were accumulated.
The 125.7 MHz 1D 13C NMR spectra of the SNase variant proteins selectively labeled with either [U-13C,15N]-Lys or [ε-13C;ε,ε-D2]-Lys were measured in D2O, H2O, and H2O : D2O (1 : 1), at 25 ∘C on a Bruker Avance 500 spectrometer equipped with a DCH cryogenic probe; simultaneous deuterium decoupling was achieved using the WALTZ16 scheme. The spectral width and repetition time were 6300 Hz and 5 s, respectively. In the experiment in H2O solution, a 4.1 mm o.d. Shigemi tube containing the protein solution was inserted into a 5 mm o.d. outer tube containing pure D2O for the internal lock signal. By taking advantage of the selective deuteration on the ε-13C in [ε-13C;ε,ε-D2]-Lys (∼ 98 at. %), the background 13C signals due to the naturally abundant, and therefore protonated, 13C nuclei were readily filtered out using the pulse scheme shown in Fig. A2.
3.1 Complete assignment of the Lys side-chain NMR signals in the SNase variant selectively labeled with SAIL-Lys
Although the chemical shifts with sequential assignments for the backbone 1H, 13C, and 15N signals of SNase are available in the BMRB (entry #16123; Chimenti et al., 2011), we reconfirmed them through the HNCA experiment for the [U-13C, 15N]-SNase variant, since the solution conditions were slightly different. The complete side-chain assignment for all 21 Lys residues was not trivial, even for the SNase variant residue selectively labeled with [U-13C, 15N]-Lys, due to the extensive signal overlap as illustrated in the F1–F3 projection of the 3D HCCH TOCSY spectrum (Fig. 1a). On the other hand, a markedly improved 3D HCCH TOCSY spectrum was obtained, under the simultaneous deuterium decoupling, for the SNase variant residue selectively labeled with SAIL-Lys (Fig. 1b), enabling us to firmly establish the full connectivity for the side-chain 1H, 13C, and15N NMR signals of the 21 Lys residues. To illustrate the improved spectral quality obtained with the SAIL-Lys in lieu of [U-13C,15N]-Lys, a panel obtained for the F1–F3 projection, along the 13C axis (F2) restricted for the chemical shift range of 40.1–45.5 ppm for the 13Cε signals, is shown for the 1Hα–1Hε2 correlation signals (Fig. 1c). By taking advantage of the well-dispersed 1Hα–1Hε2 signals, the backbone 1Hα–13Cα signals (Fig. 1e) were readily correlated to the 1Hε2–13Cε HSQC signals (Fig. 1d). Actually, all of the SAIL-Lys side-chain 13C signals were facilely and unambiguously assigned through the 3D HCCH TOCSY spectrum, yielding a complete set of the Lys side-chain NMR chemical shifts, as summarized in Table 1. It should be noted that since each one of the SAIL-Lys side-chain methylene groups (–CHD–) was stereospecifically deuterated, i.e., [U-13C,15N; β2,γ2,δ2,ε3-D4]-Lys (Fig. 1f), the Lys β3, γ3, δ3, and ε2-1H signals of the side chains of the 21 Lys residues were stereospecifically assigned. Thus, these assigned signals have the potential of providing a wealth of information on the local conformations of the Lys side chains in solution.
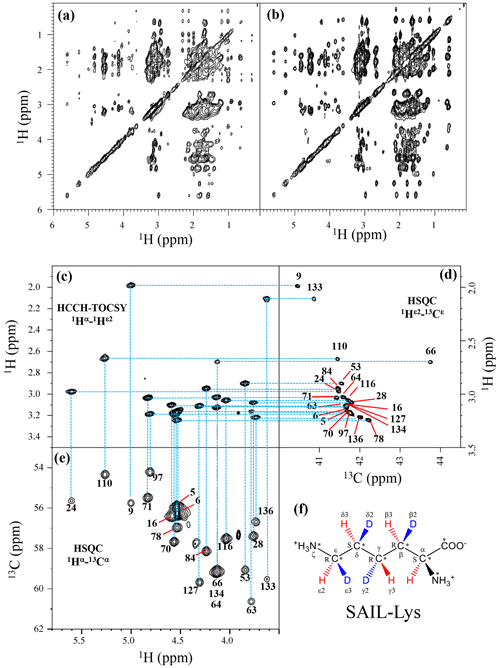
Figure 1Sequential assignment of the Lys side-chain signals for the SNase variant selectively labeled with SAIL-Lys using the 3D HCCH TOCSY experiment. Panels (a) and (b) show a comparison of the F1–F3 projections of the 3D HCCH TOCSY spectra obtained for the SNase variant selectively labeled with either [U-13C, 15N]-Lys (a) or SAIL-Lys (b). A complete side-chain signal assignment was established for the SNase variant selectively labeled with SAIL-Lys by the correlation networks on the 3D HCCH TOCSY spectrum, starting from the backbone 1Hα and 13Cα signals with assignments deposited in the BMRB (entry #16123; Chimenti et al., 2011). For example, the 1Hε2–13Cε HSQC signals in panel (d) were unambiguously correlated to the backbone 1Hα–13Cα HSQC signals in panel (e), through the 1Hα–1Hε2 correlation signals in panel (c), which represents the F1–F3 projection of the 3D HCCH TOCSY spectrum along the 13C axis (F2) restricted for the 13Cε shift range of 40.1–45.5 ppm. The structure of SAIL-Lys, [U-13C,15N; β2,γ2,δ2,ε3-D4]-Lys, is shown in panel (f). The spectrum was measured at 30 ∘C on a Bruker Avance 600 spectrometer equipped with a TXI cryogenic probe. The chemical shifts for the 1H and 13C dimensions are δTSP.
Table 1The 1H, 13C, and 15N chemical shifts for the side chains of the 21 Lys residues in Δ+PHS/V66K SNase selectively labeled with SAIL-Lys in D2O. The 1H and 13C signals were assigned using the 3D HCCH TOCSY experiment recorded on a Bruker 600 MHz spectrometer at 30 ∘C, pH 8.0. The 15N signals were assigned using a HECENZ experiment at 600 MHz (see Fig. A4). The 1H and 13C chemical shifts were referenced to TSP, while the 15N chemical shifts were referenced to DSS: δDSS−δTSP = 0.15 ppm. Since one of the prochiral methylene protons was stereospecifically deuterated in the SAIL-Lys, i.e., [U-13C,15N; β2,γ2,δ2,ε3-D4]-Lys, the observed 1H signals were unambiguously assigned. The chemical shifts for the engineered Lys-66, which has a deprotonated ζ-amino group, are shown in italics. The averaged chemical shifts are obtained by excluding Lys-66, and the measurement errors were estimated as less than 0.05 and 0.01 ppm, for the 13C and 15N and 1H chemical shifts, respectively. All chemical shifts are not corrected for the isotope shifts.
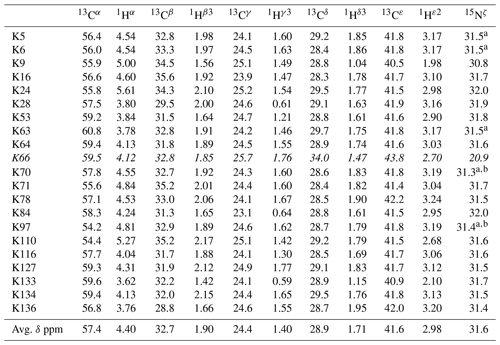
a The signals were heavily overlapped, and thus their accurate chemical shifts could not be obtained. b The 15Nζ shifts, presumably due to K70 and K97, may be reversed.
3.2 Structural information inferable from the Lys side-chain chemical shifts
Note that the chemical shifts in Table 1 for the 21 Lys residues in the SAIL-Lys-labeled SNase variant are not corrected for the various isotope-induced shifts caused by the complicated isotope-labeling pattern of the SAIL-Lys structure (see Fig. 1f). Based on comprehensive NMR data, we should be able to elucidate the dual role of the Lys side chains in terms of the conformational dynamics and functional properties of a protein in further detail, using various solution NMR methods. In this section, we briefly interpret the chemical shift data to characterize the local conformational features by the 1H, 13C, and 15N signals compiled in Table 1, which should be followed by more extensive studies in the future. Although we have not yet attempted to collect the comprehensive nuclear Overhauser effects (NOEs), such as using a fully SAIL-labeled SNase variant (Kainosho et al., 2006), it was obvious that the chemical shift data with exclusive and unambiguous assignments for the Lys residues contain an abundance of information on the side-chain conformations and ionization states of the ζ-amino groups. As described above, the unusual chemical shifts of the Lys-66 side chain confirmed the deprotonated state of its ζ-amino group. We also obtained some interesting structural information for the other Lys residues with protonated ζ-amino groups. For example, the Lys-9 side chain exists in two conformational states in the crystalline state (PDB entry #3HZX), which only differ in the χ4 angle, i.e., Form A (trans, ∼ −175∘) and Form B (gauche+, ∼ +44∘), as shown in Fig. 2a and b, respectively (see also Table A1). The significantly upfield-shifted signals observed for Lys-9 relative to the averaged chemical shifts (Δδ, ppm) are obviously due to the aromatic ring current of Tyr-93, i.e., 15Nζ (30.8 ppm, ppm), 13CHε2 ( ppm, ppm), and 1Hδ3 (1.04 ppm, ppm). These chemical shifts suggest the ζ–NH3+–π interaction, as shown by the dashed red line (Fig. 2a). Therefore, the chemical shifts for Lys-9 strongly imply that the van der Waals interactions between the aliphatic side chain, as well as the electrostatic interaction between the positively charged ζ-HN3+ and the nearby aromatic ring of Tyr-93, simultaneously contribute to preferentially stabilize the Form A conformation in solution (Fig. 2a).
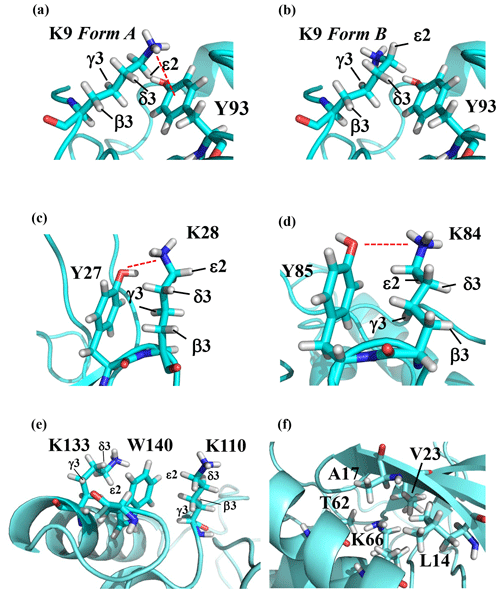
Figure 2The local structures around the Lys residues, which exhibit unusual side-chain chemical shifts, in the crystal structure of the SNase variant (PDB: 3HZX). The crystal structure of the SNase variant was solved as a complex with calcium ions and thymidine 3′,5′-diphosphate. Therefore, it may be slightly different from that in the free state. The figures were created with PyMOL 2.4 software in order to highlight the relative orientations between the Lys side chains and nearby aromatic rings (a)–(e) and Lys-66 and the surrounding hydrophobic amino acids (f). The atom nomenclature of the prochiral hydrogen atoms used in the figure is that of the IUPAC recommendations (Markley et al., 1998).
The upfield shifts of the side-chain methylenes, induced by the neighboring aromatic rings, were also detected for Lys-28, Lys-84, Lys-110, and Lys-133. Considering the local structures of Lys-28 and Lys-84 in the crystal (Fig. 2c, d), the relative orientations between Lys-28 and Tyr-27 and between Lys-84 and Tyr-85 seem to be similar to those in the crystal and are responsible for the large upfield shifts for only their 1Hγ3 signals, i.e., Lys-28: 0.61 ppm, ppm; Lys-84: 0.64 ppm, ppm, while the other 13C and 1H shifts remain within the average ranges (Table 1). The small but obvious low-field shifts for the 15Nζ (Lys-28: 31.9 ppm; Lys-84: 32.0 ppm; Δδ: +0.3 and 0.4 ppm, respectively) might be caused by the electrostatic interactions between the Oη of Tyr-27 Tyr-85 and the Nζ of Lys-28 Lys-84, respectively, as shown by the dashed red lines (Fig. 2c, d). The bulky indole ring of Trp-140 seems to simultaneously stabilize the aliphatic chains of both Lys-133 and Lys-110, inducing the upfield shifts for some of the side-chain signals, i.e., Lys-133 13CHε2 (40.9 2.10 ppm, ppm), 1Hδ3 (1.15 ppm, ppm), 1Hγ3 (0.59 ppm, ppm) and 1Hβ3 (1.42 ppm, ppm); Lys-110 1Hε2 (2.68 ppm, ppm). These upfield shifted signals indicate that the van der Waals interactions between the methylene moieties of Lys-133 and Lys-110, with the hydrophobic indole ring of Trp-140 sandwiched in the middle, are also preserved in solution (Fig. 2e). Interestingly, the chemical shift differences between the two prochiral protons attached to the ε-carbons, observed for the SNase variant residue selectively labeled with [U-13C,15N]-Lys, of Lys residues 110 and 133 are considerably larger than those of the other 19 Lys residues, which are much smaller than ∼ 0.05 ppm (Fig. A3). Since the 1Hε2 chemical shifts were observed at a 0.15 and 0.17 ppm higher field than the 1Hε3 chemical shifts for Lys-110 and -133, respectively, the conformations of these two Lys residues are likely to be similar to those in the crystal (Fig. 2e).
On the other hand, the unusual chemical shifts observed for the Lys-66 residue, which is trapped within the hydrophobic environment engineered in the engineered SNase variant (Fig. 2f), clearly reveal the strong influence of the ionization state of the ζ-amino group on the Lys side chain. As shown in Table 1, the 15Nζ chemical shift of the ζ-ND2 of Lys-66 in the SNase variant appears at an unusually upfield position, as compared to the averaged chemical shift range for the ζ-ND3+ in the other Lys residues, i.e., 15Nζ (Lys-66: 20.9 ppm, ppm), which is close to the value of the ζ-NH2 chemical shift, 23.3 ppm, previously reported for Lys-66 in the [U-13C,15N]-SNase variant (André et al., 2007; Takayama et al., 2008). Evidently, the 15Nζ chemical shifts provide an unambiguous clue to distinguish between the deprotonated and protonated ζ-amino groups of Lys residues. However, the complete side-chain assignment including the terminal ζ-15N signals through conventional methods using a [U-13C,15N] protein is usually laborious and occasionally not practical.
In comparison with charged Lys side chains, deprotonation of the ζ-amino group of Lys-66 is characterized by sizable 1H and 13C chemical shift differences, i.e., 13CHε2 ( ppm, ppm), 13CHδ3 ( ppm, ppm), and 13CHγ3 ( ppm, ppm). These deprotonation shifts, in particular, those of 13Cε and/or 13Cδ, could therefore be used as unambiguous indices to characterize the ionization states of the ζ-amino groups of Lys residues in a protein, since they can be accurately and readily observed and assigned using a protein selectively labeled with SAIL-Lys. It should be noted, however, that the side-chain chemical shifts in general might significantly vary according to the local environments, such as the relative position to aromatic rings, and thus the results obtained exclusively from the side-chain chemical shifts might not be absolutely reliable. To avoid any possible uncertainties in characterizing the ionization states of ζ-amino groups, an alternative approach using the deuterium-induced isotope shifts of the SAIL-Lys side-chain 13C signals may be considered.
3.3 Characterization of the ionization state of the ζ-amino group of Lys residues using the effects of deuterium-induced isotope shifts on the side-chain 13C and 15N signals
In our previous studies investigating the effects of the deuterium-induced isotope shifts on the 13C signals adjacent to polar functional groups with an exchangeable hydrogen, such as hydroxyl (OH) or sulfhydryl (SH) groups, we demonstrated that those isotope shifts are versatile indices for identifying residues, such as Tyr, Thr, Ser, or Cys, with exceptionally slow hydrogen exchange rates (Takeda et al., 2014). For example, in a protein selectively labeled with [ζ-13C]-Tyr, the Tyr residues have much slower hydrogen exchange rates for the η-hydroxyl groups than the isotope shift differences in the 13Cζ signals and exhibit well-resolved pairwise signals with nearly equal intensities in the 1D 13C NMR spectrum in H2O : D2O (1 : 1) (Takeda et al., 2009). The up- and low-field counterparts of the pairwise 13Cζ signals correspond to those in D2O and H2O, respectively, and their relative intensities reflect the fractionation factors, i.e., [OD] [OH]. Similar approaches have been developed for Ser, Thr, and Cys residues, using the 13Cβ signals observed for proteins selectively labeled with [β-13C; β, β-D2]-Ser, [β-13C; β-D]-Thr, and [β-13C; β, β-D2]-Cys, respectively (Takeda et al., 2010, 2011). Since the isolated 13Cβ(D2) or 13Cβ(D) moieties in the labeled amino acids give extremely narrow signals under the deuterium decoupling, the 13C NMR signals can be obtained with remarkably high sensitivities, especially with a 13C direct observing cryogenic probe. Interestingly, while the fractionation factors for the Ser and Thr hydroxyl groups, i.e., [OD] [OH], are usually close to unity, as also for the Tyr residues, those for the Cys sulfhydryl groups, i.e., [SD] [SH], are around 0.4–0.5 (Takeda et al., 2010, 2011). The methods are especially important, since the functional groups of the residues readily identified as having exceptionally slow hydrogen exchange rates are most likely to be involved in hydrogen bonding networks and/or located in distinctive local environments.
Although the idea of estimating the hydrogen exchange rates by the deuterium-induced isotope shifts on the 13C nuclei adjacent to functional groups with exchangeable hydrogens was originally exploited years ago, for the backbone amide groups in the selectively labeled proteins with [C'-13C]-amino acid(s) (Kainosho and Tsuji, 1982; Markley and Kainosho, 1993), it has not yet been applied for the Lys ζ-amino groups. Having established the complete assignment for the 21 Lys residues in the SNase variant selectively labeled with SAIL-Lys (Table 1), we next examined the deuterium-induced chemical shifts in detail for the Lys side-chain signals. In the case of Lys residues, the NMR signals of the ζ-amino 15N and ε- or δ-carbon 13C signals would be plausible candidates for probing the deuterium substitution effects. There have several reports on the isotope shifts of the δ- and ε-13C for the Lys residues induced by the deuteration of ζ-amino groups (Ladner et al., 1975; Led and Petersen, 1979; Hansen, 1983; Dziembowska et al., 2004; Tomlinson et al., 2009; Platzer et al., 2014). However, it seems no comprehensive studies have applied the deuterium-induced isotope shifts on 13Cε signals to characterize the ionization states of Lys residues.
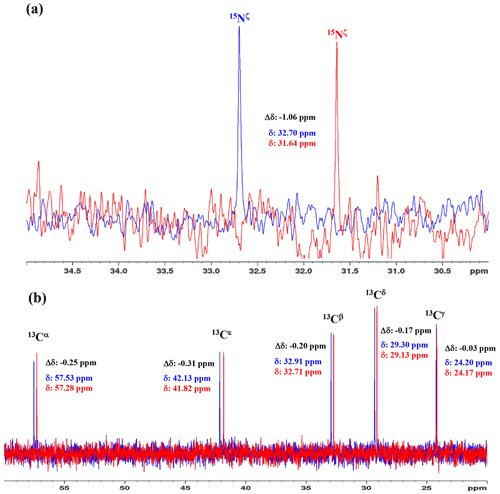
Figure 31D 15N and 13C NMR spectra of [15N2]-lysine in H2O and D2O. The 96.3 MHz 1D 15N NMR spectra (a) and 239.0 MHz 1D 13C NMR spectra (b) of [15N2]-lysine were measured at 30 ∘C on a Bruker Avance III 950 spectrometer with a TCI cryogenic probe, using ∼ 70 mM solutions of 20 mM tris buffer prepared with H2O (or D2O) at pH (or pD) 8.0. The NMR spectra and the chemical shifts, δDSS (13C 15N), shown in blue and red, are those for the H2O and D2O buffer solutions, respectively. The deuterium-induced shifts, ΔδDSS ppm : δ (in D2O) − δ (in H2O), for the 15Nζ and side-chain 13C signals are shown in black.
We first examined the 1D 13C and 15N NMR spectra of [15N2]-Lys in D2O and H2O, at pH 8 and 30 ∘C, to choose the suitable NMR probes to distinguish between the deprotonated and protonated ζ-amino groups (Fig. 3). The ζ-15N signal appears at ∼ 1 ppm upfield in D2O relative to that in H2O (Fig. 3a), and the aliphatic 13C signals of [15N2]-Lys at the natural abundance also showed isotope shifts, Δδ[13Ci (in D2O)-δ13Ci (in H2O)], i.e., 13Cα, −0.25 ppm; 13Cβ, −0.20 ppm; 13Cγ, −0.03 ppm; 13Cδ, −0.17 ppm; and 13Cε, −0.31 ppm (Fig. 3b). Although the isotope shifts for 13Cα and 13Cβ are due to the deuteration of the α-amino group, those for 13Cδ and 13Cε are obviously due to the deuteration of the ζ-amino group. Considering the finding that the 13Cε of Lys gives an isolated signal far from the others and exhibits a ∼ 1.8-fold larger isotope shift as compared to 13Cδ, the 13Cε and 15Nζ signals seem to be good candidates for probing the ionization states of Lys residues in the SNase variant.
Table 2Deuterium-induced isotope shifts for the side-chain 15Nζ and 13Cε signals of the 21 Lys residues in Δ+PHS/V66K SNase. The 15Nζ chemical shift (referenced to DSS) and 13Cε chemical shift (referenced to TSP) data in H2O and D2O were obtained for the SNase labeled with either SAIL-Lys or [ε-13C;ε,ε-D2]-Lys, respectively. Note that in the 1D 13Cε data measured at 125.7 MHz, the 1D 13C spectra have much higher precision as compared to those obtained using the 2D HSQC using the SNase labeled with SAIL-Lys. Spectra were recorded on a Bruker 600 MHz spectrometer at 30 ∘C, pH 8.0. The averaged chemical 13C and 15N chemical shifts in the last row were obtained for the Lys residues with protonated ζ-amino groups, except for Lys-66 (italics) which has a deprotonated ζ-amino group. Although the exact chemical shifts could not be obtained for the residues shown by “–”, the deuterium-induced 15N and 13C shifts were almost the same as those of the other residues, except for Lys-66. The averaged Δδ values show the differences between the averaged 15Nζ and 13Cε, except for Lys-66, which are the differences between the 15Nζ and 13Cε shifts in H2O and D2O. Negative Δδ values indicate the chemical shifts in D2O are upfield shifted due to the deuteration of the ζ-amino groups.
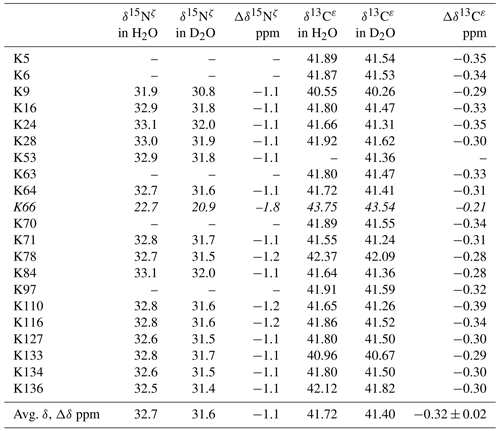
Although the 15Nζ and 13Cε chemical shifts for the Lys residues can be measured by the HECENZ and 1H–13C ct (constant time) HSQC experiments, respectively, using the SNase variant selectively labeled with [U-13C,15N]-Lys or SAIL-Lys, it was rather difficult to determine the accurate isotope shifts of the 15Nζ and 13Cε signals for all 21 Lys residues using these methods. In particular, the accurate chemical shift measurement for an individual 13Cε signal was hampered by the poor quality of the ct HSQC spectrum, even for the protein labeled with SAIL-Lys (Fig. A3). Therefore, we used [ε-13C; ε,ε-D2]-Lys to reduce the line widths of the 13Cε signals for the Lys residues in the SNase variant. As expected, the 1D 13C NMR spectra of the SNase variant selectively labeled with [ε-13C;ε,ε-D2]-Lys showed remarkably well-resolved signals, with line widths less than 2 Hz, under the 1H 2D double decoupling conditions (Fig. 4). Note that the weak background signals due to the naturally abundant 13C nuclei were filtered out in this spectrum (Fig. A2). By referring to the chemical shifts in Table 1, which were determined using the 3D HCCH TOCSY experiment for the SNase labeled with SAIL-Lys, all of the 1-D 13Cε signals were unambiguously assigned (Fig. 4a, b). The chemical shifts of 13Cε are slightly different among the data sets, because the isotope shifts induced by the nearby isotopes on the 13Cε signals are different for SAIL-Lys and [ε-13C;ε,ε-D2]-Lys (Tables 1, 2). The 13Cε chemical shifts in H2O and D2O, which were accurately determined by the 1D 13C NMR spectra, are presented in Fig. 5. At a glance, the 13Cε spectra in Fig. 5a and c look almost the same, since the signals moved upfield with a constant increment of ppm, except for the 13Cε signal of Lys-66 (Table 2). Since the δ13Cε values in H2O and D2O are very close to those for the free [15N2]-Lys (Fig. 3b), the ζ-amino groups are protonated in H2O and deuterated in D2O, and thus the averaged deuterium-induced isotope shift was designated as Δδ13Cε [NζD-NζH]. Similarly, the averaged Δδ15Nζ [NζD-NζH], excluding the value for Lys-66, was determined to be ppm (Williamson et al., 2013), which was also close to the free [15N2]-Lys (Fig. 3a). The Δδ13Cε and Δδ15Nζ for Lys-66, which are −0.21 and −1.8 ppm (Table 2), respectively, confirmed that the ζ-amino group of this residue is deprotonated at pH 8 and should be designated as Δδ13Cε [NζD2-NζH2] and Δδ15Nζ [NζD2-NζH2]. Interestingly, the fact that the averaged Δδ13Cε [NζD-NζH], −0.32 ppm, was ∼ 1.5 times larger than the Δδ13Cε [NζD2-NζH2] for Lys-66, −0.21 ppm, might suggest that the deuterium-induced isotope shift on 13Cε is proportional to the number of hydrogen atoms on the ζ-amino groups. In contrast, the averaged Δδ15Nζ [NζD-NζH], −1.1 ppm, was much smaller than that of the Δδ15Nζ [NζD2-NζH2] for Lys-66, −1.8 ppm.
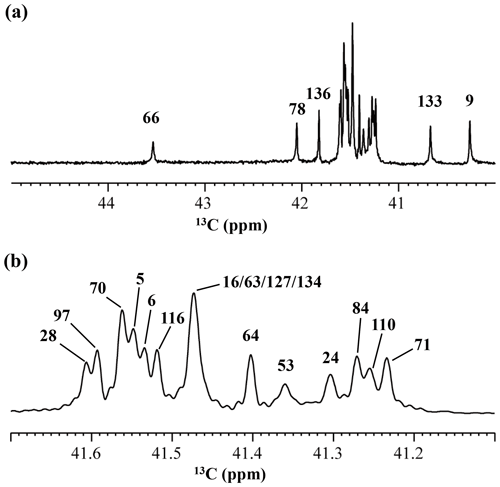
Figure 4125.7 MHz {1H, 2D}-decoupled 1D 13C NMR spectrum for the SNase variant selectively labeled with [ε-13C;ε,ε-D2]-Lys. The spectra were measured at 25 ∘C, pH 8.0, in D2O solution on an Avance 500 spectrometer equipped with a DCH cryogenic probe. Although only a few discrete 13Cε signals are apparent in panel (a), the congested spectral region around 41–42 ppm shows well-separated signals due to their narrow line widths of 1–2 Hz (b). All of the 1D NMR signals for 13Cε were readily assigned using the chemical shift data, δTSP (13C) obtained from the 3D HCCH TOCSY experiment for the SNase variant selectively labeled with SAIL-Lys (see Sect. 3.1).
We also measured the 1D 13C NMR spectrum of the SNase variant selectively labeled with [ε-13C;ε,ε-D2]-Lys in H2O : D2O (1 : 1), to search for the Lys residues with slowly exchanging ζ-amino groups. Clearly, there are no such residues in the SNase variant at pH 8 and 30 ∘C, as shown in Fig. 5b. Due to the rapid hydrogen exchange rates for all 21 Lys residues in this protein, the observed isotope shifts on 13Cε were exactly half of the Δδ13Cε [NζD2-NζH2] for Lys-66 or Δδ13Cε [NζD-NζH] for the rest of the Lys residues. The hydrogen exchange rate constant (kex) for the ζ-amino group of Lys-66 in the SNase variant, which is deeply embedded in the hydrophobic cavity originally occupied by Val-66 in the wild-type SNase, was 93±5 s−1 at pH 8 and −1 ∘C (Takayama et al., 2008). Therefore, the hydrogens on the ζ-amino groups in all 21 Lys residues in the SNase variant are rapidly exchanging, and thus the observed chemical shifts for the 13Cε of Lys-66 and the rest of the Lys residues in H2O : D2O (1 : 1) are the time averages for three isotopomers, NH2, NHD, and ND2, with nearly a 1 : 2 : 1 ratio for Lys-66, and for four isotopomers, NH, NH2D+, NHD, and ND, with a ratio of 1 : 3 : 3 : 1. Since the time-averaged signals for Lys-66 and other Lys residues in H2O : D2O (1 : 1) appeared exactly in the middle of the spectra observed in H2O and D2O (Fig. 5a, c), the fractional factors for the isotopomers are nearly identical, as statistically random distributions.
In this article, we have shown that comprehensive NMR information can be obtained using cutting-edge isotope-aided NMR technologies for the Lys side-chain moieties, comprising a long hydrophobic methylene chain and a hydrophilic ζ-amino group, to facilitate hitherto unexplored investigations toward elucidating the dual nature of the Lys residues in a protein. The unambiguously assigned 13C signals, together with the stereospecifically assigned prochiral protons for each of the long consecutive methylene chains, which first became available using the stereo-array isotope labeling (SAIL) method, provide unprecedented opportunities to examine the conformational features around the Lys residues in detail. The ionization states of the ζ-amino groups of Lys residues, which play crucial roles in the biological functions of proteins, could be readily characterized by the deuterium-induced isotope shifts on the ε-13C signals observed by the 1D 13C NMR spectroscopy of a protein selectively labeled with [ε-13C;ε,ε-D2]-Lys. Both methods should work equally well for larger proteins, for which previous NMR approaches were rarely applicable. Therefore, these methods will contribute toward clarifying the structural and functional roles of the Lys residues in biologically important proteins.
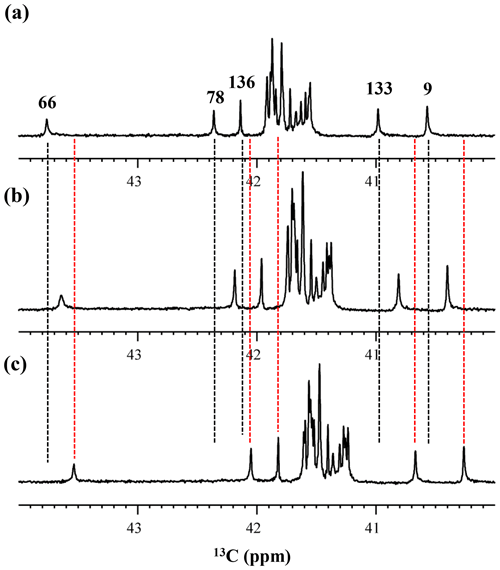
Figure 5Isotope shifts on the 13Cε signals of the Lys residues in the SNase variant selectively labeled with [ε-13C;ε,ε-D2]-Lys, caused by the deuterium substitutions for the ζ-amino groups. The 125.7 MHz {1H, 2D}-decoupled 1D 13C NMR spectra were measured at 25 ∘C, pH 8.0, in either H2O (a), H2O : D2O (1 : 1) (b), or D2O (c) solutions on an Avance 500 spectrometer equipped with a DCH cryogenic probe in H2O (a), H2O : D2O (1 : 1) (b), and D2O (c) solutions. The vertical dotted black and red lines show the chemical shifts observed in 100 % H2O and D2O, respectively. The complete data for the deuterium-induced isotope shifts for the side-chain 15Nζ and 13Cε signals are summarized in Table 2.
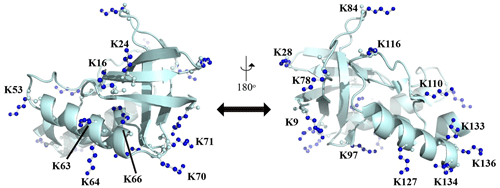
Figure A1Distribution of the Lys residues in the crystalline structure of the Δ+PHS/V66K variant of SNase (PDB#: 3HZX). All of the side-chain moieties of the Lys residues, which are shown by the ball-and-stick model in blue, exist on the protein surface, except for the Lys-66 (K66). This engineered residue is locked in the protein interior that is originally occupied by the Val side chain in the wild-type protein. Two Lys residues, K5 and K6, were not visible in the X-ray analysis of the SNase complexed with calcium and thymidine 3′,5′-diphosphate, and thus it may be slightly different from that in the free state. The figure was created using PyMOL 2.4 software.
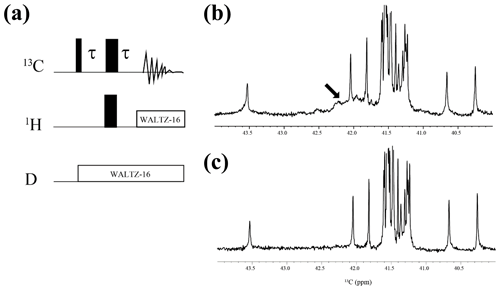
Figure A2{1H, 2D}-1D 13C NMR spectra for the SNase variant selectively labeled with [ε-13C;ε,ε-D2]-Lys. The 125.7 MHz 13C NMR spectra were measured at 25 ∘C on a Bruker Avance 500 spectrometer equipped with a 13C-observing DCH cryogenic probe. The broad background signals observed in the spectrum (b), indicated by a thick arrow, are due to the natural abundant 13C atoms bound to proton(s), which are readily filtered out to give the spectrum (c), using the pulse scheme shown in panel (a). The narrow and wide bars in the scheme represent 90 and 180∘ rectangular pulses, respectively, and are applied along the x axis at τ=1.7 ms, which corresponds to 1JCH. The SNase variant was dissolved in 100 % D2O buffer, containing 20 mM sodium phosphate and 100 mM potassium chloride at pH 8.0. 13C chemical shifts are referenced to TSP.
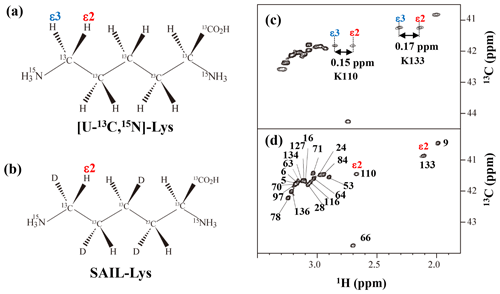
Figure A3Comparison between the 13Cε regions of the 2D 1H–13C constant time (ct) HSQC spectra obtained for the SNase variant selectively labeled with [U-13C,15N]-Lys (a) and [U-13C,15N;β2,γ2,δ2,ε3-D4]-Lys, SAIL-Lys (b). Since ε-carbons for the [U-13C,15N]-Lys residues are attached to the two prochiral protons, 1Hε2 and 1Hε3, a pairwise correlation signals, namely 1Hε2–13Cε and 1Hε3–13Cε, can be observed for each of the ε-carbons (c). However, considerable large chemical shift differences between the prochiral ε-methylene protons were observed only for K110 (Δδ, 0.15 ppm) and for K133 (Δδ, 0.17 ppm), and the other 19 Lys residues showed differences less than ∼ 0.05 ppm. On the other hand, ε-carbons for the SAIL-Lys residues are attached only to the ε2-protons, and all of the correlation signals are automatically assigned to 1Hε2 (d). Cε peaks are labeled with their assignment. The spectra were measured at 30 ∘C on an Avance 600 spectrometer equipped with a TXI cryogenic probe. 1H and 13C chemical shifts are referenced to TSP: δDSS–δTSP = 0.15 ppm.
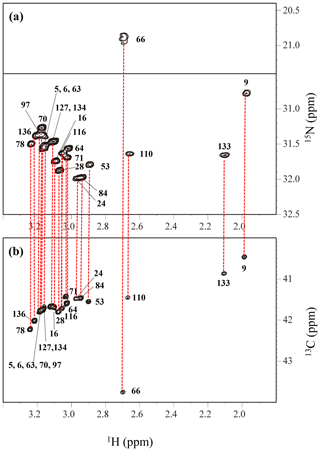
Figure A4The HECENZ (a) and ct HSQC (b) spectra, recorded on a Bruker Avance 600 spectrometer (1H, 600.0 MHz; 13C, 150.9 MHz; 15N, 60.8 MHz) using 1.4 mM D2O solutions of the Δ+PHS/V66K SNase variants selectively labeled with SAIL-Lys, at 30 ∘C, pD 8.0. Note that the chemical shifts of the 1H and 13C dimensions are referenced to DSS, while the 15N dimension is referenced to TSP: δDSS–δTSP = 0.15 ppm. The detailed experimental parameters are discussed in Sect. 2.2.
All of the NMR data supporting this work are shown in the paper. Isotopically labeled lysines are available on request from Taiyo Nippon Sanso Co. at https://stableisotope.tn-sanso.co.jp (last access: 23 April 2021).
MT prepared the isotopically labeled protein samples, collected and analyzed NMR data, and wrote the paper. YM measured and analyzed high-field NMR spectra. TT prepared isotopically labeled lysines. MK supervised the project and edited the paper.
The authors declare that they have no conflict of interest.
This article is part of the special issue “Robert Kaptein Festschrift”. It is not associated with a conference.
This work was performed, in part, using the NMR spectrometers with the ultra-high magnetic fields under the Collaborative Research program of the Institute for Protein Research, Osaka University (grant nos. NMRCR-19-05 and NMRCR-20-05).
This research has been supported by Grants-in-Aid in Innovative Areas (grant nos. 21121002 and 26119005) and also in part by the Kurata Memorial Hitachi Science and Technology Foundation and by Grants-in-Aid for Scientific Research (grant no. 25440018).
This paper was edited by Rolf Boelens and reviewed by three anonymous referees.
André, I., Linse, S., and Mulder, F. A.: Residue-specific pKa determination of lysine and arginine side chains by indirect 15N and 13C NMR spectroscopy: application to apo calmodulin, J. Am. Chem. Soc., 129, 15805–15813, https://doi.org/10.1021/ja0721824, 2007.
Barbas III, C. F., Heine, A., Zhong, G., Hoffmann, T., Gramatikova, S., Björnestedt, R., List, B., Anderson, J., Stura, E. A., Wilson, I. A., and Lerner, R. A.: Immune versus natural selection: antibody aldolases with enzymic rates but broader scope, Science, 278, 2085–2092, https://doi.org/10.1126/science.278.5346.2085, 1997.
Cavanagh, J., Fairbrother, W. J., Palmer, A. G., Rance, M., and Skelton, J. J.: Protein NMR Spectroscopy: Principles and Practice, Academic Press, New York, USA, 2007.
Chimenti, M. S., Castañeda, C. A., Majumdar, A., and García-Moreno, E. B.: Structural origins of high apparent dielectric constants experienced by ionizable groups in the hydrophobic core of a protein, J. Mol. Biol., 405, 361–377, https://doi.org/10.1016/j.jmb.2010.10.001, 2011.
Clore, G. M., Bax, A., Driscoll, P. C., Wingfield, P. T., and Gronenborn, A. M.: Assignment of the side chain 1H and 13C resonances of interleukin-1 beta using double- and triple-resonance heteronuclear three-dimensional NMR spectroscopy, Biochemistry, 29, 8172–8184, https://doi.org/10.1021/bi00487a027, 1990.
Damblon, C., Raquet, X., Lian, L. Y., Lamotte-Brasseur, J., Fonze, E., Charlier, P., Roberts, G. C., and Frère, J. M.: The catalytic mechanism of beta-lactamases: NMR titration of an active-site lysine residue of the TEM-1 enzyme, Proc. Natl. Acad. Sci. USA, 93, 1747–1752, https://doi.org/10.1073/pnas.93.5.1747, 1996.
Dziembowska, T., Hansen, P. E., and Rozwadowski, Z.: Studies based on deuterium isotope effect on 13C chemical shifts, Prog. Nucl. Magn. Reson. Spectrosc., 45, 1–29, https://doi.org/10.1016/j.pnmrs.2004.04.001, 2004.
Farmer II, B. T. and Venters, R. A.: Assignment of aliphatic side chain 1HN 15N resonances in perdeuterated proteins, J. Biomol. NMR, 7, 59–71, https://doi.org/10.1007/BF00190457, 1996.
Fitch, C. A., Karp, D. A., Lee, K. K., Stites, W. E., Lattman, E. E., and García-Moreno, E. B.: Experimental pK(a) values of buried residues: analysis with continuum methods and role of water penetration, Biophys. J., 82, 3289–3304, https://doi.org/10.1016/s0006-3495(02)75670-1, 2002.
Gao, G., Prasad, R., Lodwig, S. N., Unkefer, C. J., Beard, W. A., Wilson, S. H., and London, R. E.: Determination of lysine pK values using [5-13C]lysine: application to the lyase domain of DNA Pol beta, J. Am. Chem. Soc., 128, 8104–8105, https://doi.org/10.1021/ja061473u, 2006.
García-Moreno, B., Dwyer, J. J., Gittis, A. G., Lattman, E. E., Spencer, D. S., and Stites, W. E.: Experimental measurement of the effective dielectric in the hydrophobic core of a protein, Biophys. Chem., 64, 211–224, https://doi.org/10.1016/S0301-4622(96)02238-7, 1997.
Hansen, P. E.: Isotope effects on nuclear shielding, Annu. Rep. NMR Spectrosc., 15, 105–234, 1983.
Harris, T. K. and Turner, G. J.: Structural basis of perturbed pKa values of catalytic groups in enzyme active sites, IUBMB Life, 53, 85–98, https://doi.org/10.1080/15216540211468, 2002.
Highbarger, L. A., Gerlt, J. A., and Kenyon, G. L.: Mechanism of the reaction catalyzed by acetoacetate decarboxylase. Importance of lysine 116 in determining the pKa of active-site lysine 115, Biochemistry, 35, 41–46, https://doi.org/10.1021/bi9518306, 1996.
Isom, D. G., Cannon, B. R., Castaneda, C. A., Robinson, A., and Garcia-Moreno, B.: High tolerance for ionizable residues in the hydrophobic interior of proteins, Proc. Natl. Acad. Sci. USA., 105, 17784–17788, https://doi.org/10.1073/pnas.0805113105, 2008.
Iwahara, J., Jung, Y. S., and Clore, G. M.: Heteronuclear NMR spectroscopy for lysine NH3 groups in proteins: unique effect of water exchange on 15N transverse relaxation, J. Am. Chem. Soc., 129, 2971–2980, https://doi.org/10.1021/ja0683436, 2007.
Kainosho, M. and Tsuji, T. Assignment of the three methionyl carbonyl carbon resonances in Streptomyces subtilisin inhibitor by a carbon-13 and nitrogen-15 double-labeling technique. A new strategy for structural studies of proteins in solution, Biochemistry, 21, 6273–6279, https://doi.org/10.1021/bi00267a036, 1982.
Kainosho, M., Torizawa, T., Iwashita, Y., Terauchi, T., Ono, A. M., and Güntert, P.: Optimal isotope labelling for NMR protein structure determinations, Nature, 440, 52–57, https://doi.org/10.1038/nature04525, 2006.
Kesvatera, T., Jönsson, B., Thulin, E., and Linse, S.: Measurement and modelling of sequence-specific pKa values of lysine residues in calbindin D9k, J. Mol. Biol., 259, 828–839, https://doi.org/10.1006/jmbi.1996.0361, 1996.
Ladner, H. K., Led, J. J., and Grant D. M.: Deuterium isotope effects on 13C chemical shifts in amino acids and dipeptides, J. Magn. Reson., 20, 530–534, https://doi.org/10.1016/0022-2364(75)90010-4, 1975.
Led, J. J. and Petersen, S. B.: Deuterium isotope effects on carbon-13 chemical shifts in selected amino acids as function of pH, J. Magn. Reson., 33, 603–617, https://doi.org/10.1016/0022-2364(79)90172-0, 1979.
Liepinsh, E. and Otting, G.: Proton exchange rates from amino acid side chains-implications for image contrast, Magn. Reson. Med., 35, 30–42, https://doi.org/10.1002/mrm.1910350106, 1996.
Liepinsh, E., Otting, G., and Wüthrich, K.: NMR spectroscopy of hydroxyl protons in aqueous solutions of peptides and proteins, J. Biomol. NMR, 2, 447–465, https://doi.org/10.1007/BF02192808, 1992.
Markley, J. L. and Kainosho, M.: Stable isotope labeling and resonance assignments in larger proteins, in: NMR of Macromolecules, Oxford University Press, Oxford, UK, 101–152, 1993.
Markley, J. L., Bax, A., Arata, Y., Hilbers, C. W., Kaptein, R., Sykes, B. D., Wright, P. E., and Wüthrich, K.: Recommendations for the presentation of NMR structures of proteins and nucleic acids, Eur. J. Biochem., 256, 1–15, https://doi.org/10.1046/j.1432-1327.1998.2560001.x, 1998.
Otting, G. and Wüthrich, K.: Studies of protein hydration in aqueous solution by direct NMR observation of individual protein-bound water molecules, J. Am. Chem. Soc., 111, 1871–1875, https://doi.org/10.1021/ja00187a050, 1989.
Otting, G., Liepinsh, E., and Wüthrich, K.: Proton exchange with internal water molecules in the protein BPTI in aqueous solution, J. Am. Chem. Soc., 113, 4363–4364, https://doi.org/10.1021/ja00011a068, 1991.
Platzer, G., Okon, M., and McIntosh, L. P.: pH-Dependent random coil 1H, 13C, and 15N chemical shifts of the ionizable amino acids: a guide for protein pKa measurements, J. Biomol. NMR, 60, 109–129, https://doi.org/10.1007/s10858-014-9862-y, 2014.
Poon, D. K., Schubert, M., Au, J., Okon, M., Withers, S. G., and McIntosh, L. P.: Unambiguous determination of the ionization state of a glycoside hydrolase active site lysine by 1H-15N heteronuclear correlation spectroscopy, J. Am. Chem. Soc., 128, 15388–15389, https://doi.org/10.1021/ja065766z, 2006.
Segawa T., Kateb F., Duma L., Bodenhausen G., and Pelupessy P.: Exchange rate constants of invisible protons in proteins determined by NMR spectroscopy, ChemBioChem, 9, 537–542, https://doi.org/10.1002/cbic.200700600, 2008.
Stites, W. E., Gittis, A. G., Lattman, E. E., and Shortle, D.: In a staphylococcal nuclease mutant the side chain of a lysine replacing valine 66 is fully buried in the hydrophobic core, J. Mol. Biol., 221, 7–14, https://doi.org/10.1016/0022-2836(91)80195-z, 1991.
Takayama, Y., Castañeda, C. A., Chimenti, M., García-Moreno, B., and Iwahara, J.: Direct evidence for deprotonation of a lysine side chain buried in the hydrophobic core of a protein, J. Am. Chem. Soc., 130, 6714–6715, https://doi.org/10.1021/ja801731g, 2008.
Takeda, M., Jee, J., Ono, A. M., Terauchi, T., and Kainosho, M.: Hydrogen exchange rate of tyrosine hydroxyl groups in proteins as studied by the deuterium isotope effect on Cζ chemical shifts, J. Am. Chem. Soc., 131, 18556–18562, https://doi.org/10.1021/ja907911y, 2009.
Takeda, M., Jee, J., Terauchi, T., and Kainosho, M.: Detection of the sulfhydryl groups in proteins with slow hydrogen exchange rates and determination of their proton/deuteron fractionation factors using the deuterium-induced effects on the 13Cβ NMR signals, J. Am. Chem. Soc., 132, 6254–6260, https://doi.org/10.1021/ja101205j, 2010.
Takeda, M., Jee, J., Ono, A. M., Terauchi, T., and Kainosho, M.: Hydrogen exchange study on the hydroxyl groups of serine and threonine residues in proteins and structure refinement using NOE restraints with polar side chain groups, J. Am. Chem. Soc., 133, 17420–17427, https://doi.org/10.1021/ja206799v, 2011.
Takeda, M., Miyanoiri, Y., Terauchi, T., Yang, C.-J., and Kainosho, M.: Use of H/D isotope effects to gather information about hydrogen bonding and hydrogen exchange rates, J. Magn. Reson., 241, 148–154, https://doi.org/10.1016/j.jmr.2013.10.001, 2014.
Terauchi, T., Kamikawai, T., Vinogradov, M. G., Starodubtseva, E. V., Takeda, M., and Kainosho, M.: Synthesis of stereoarray isotope labeled (SAIL) lysine via the “head-to-tail” conversion of SAIL glutamic acid, Org. Lett., 13, 161–163, https://doi.org/10.1021/ol1026766, 2011.
Tomlinson, J. H., Ullah, S., Hansen, P. E., and Williamson, M. P.: Characterization of Salt bridges to Lysines in the Protein G B1 Domain, J. Am. Chem. Soc., 131, 4674–4684, https://doi.org/10.1021/ja808223p, 2009.
Torchia, D. A., Sparks, S. W., and Bax, A.: Staphylococcal nuclease: Sequential assignments and solution structure, Biochemistry, 28, 5509–5524, https://doi.org/10.1021/bi00439a028, 1989.
Williamson, M. P., Hounslow, A. M., Ford, J., Fowler, K., Hebditch, M., and Hansen, P. E.: Detection of salt bridges to lysines in solution in barnase, Chem. Commun., 49, 9824–9826, https://doi.org/10.1039/c3cc45602a, 2013.