the Creative Commons Attribution 4.0 License.
the Creative Commons Attribution 4.0 License.
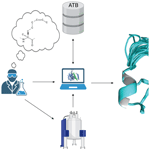
Facilitating the structural characterisation of non-canonical amino acids in biomolecular NMR
Sarah Kuschert
Martin Stroet
Yanni Ka-Yan Chin
Anne Claire Conibear
Xinying Jia
Thomas Lee
Christian Reinhard Otto Bartling
Kristian Strømgaard
Peter Güntert
Karl Johan Rosengren
Alan Edward Mark
Peptides and proteins containing non-canonical amino acids (ncAAs) are a large and important class of biopolymers. They include non-ribosomally synthesised peptides, post-translationally modified proteins, expressed or synthesised proteins containing unnatural amino acids, and peptides and proteins that are chemically modified. Here, we describe a general procedure for generating atomic descriptions required to incorporate ncAAs within popular NMR structure determination software such as CYANA, CNS, Xplor-NIH and ARIA. This procedure is made publicly available via the existing Automated Topology Builder (ATB) server (https://atb.uq.edu.au, last access: 17 February 2023) with all submitted ncAAs stored in a dedicated database. The described procedure also includes a general method for linking of side chains of amino acids from CYANA templates. To ensure compatibility with other systems, atom names comply with IUPAC guidelines. In addition to describing the workflow, 3D models of complex natural products generated by CYANA are presented, including vancomycin. In order to demonstrate the manner in which the templates for ncAAs generated by the ATB can be used in practice, we use a combination of CYANA and CNS to solve the structure of a synthetic peptide designed to disrupt Alzheimer-related protein–protein interactions. Automating the generation of structural templates for ncAAs will extend the utility of NMR spectroscopy to studies of more complex biomolecules, with applications in the rapidly growing fields of synthetic biology and chemical biology. The procedures we outline can also be used to standardise the creation of structural templates for any amino acid and thus have the potential to impact structural biology more generally.
- Article
(3561 KB) - Full-text XML
- BibTeX
- EndNote
The 20 genetically encoded amino acids, together with selenocysteine and pyrrolysine, provide the basis for most proteins and peptides that make up the machinery of life (Liu and Schultz, 2010; Huang et al., 2010; Bullwinkle et al., 2014). The use of just 22 amino acids, however, limits the structural complexity and functional diversity that can be achieved. The chemical and functional diversity of ribosomally synthesised proteins is further expanded by post-translational modification (PTM), including processes such as acylation, methylation, phosphorylation, oxidation and epimerisation (Aebersold et al., 2018; Barber and Rinehart, 2018; Walsh et al., 2005). Non-ribosomal synthesis pathways also expand chemical diversity, leading to the production of typically short peptides containing non-canonical amino acids (ncAAs), as well as backbone or side-chain cyclisation (Link et al., 2003; Tharp et al., 2020; Caboche et al., 2008; Goodrich and Frueh, 2015; Martínez-Núñez and López, 2016; Strieker et al., 2010). Such non-ribosomal peptides (NRPs) are prevalent in bacteria and fungi, which produce a wide range of bioactive peptides (Caboche et al., 2008; Marahiel, 2009). In addition to pathways found in nature, chemical synthesis, enzymatic modification, genetic code expansion and site-selective biorthogonal transformations are increasingly used to introduce novel ncAAs and PTMs into peptides and proteins (Bondalapati et al., 2016; Chuh et al., 2016; Conibear, 2020; Hoyt et al., 2019; Thompson and Muir, 2020) for both investigations of peptide structure and function as well as in the design and optimisation of pharmaceuticals (Noren et al., 1989; Coin, 2018; Hoesl and Budisa, 2011; Johnson et al., 2010; Wang et al., 2001, 2020; Zou et al., 2018).
Despite the prevalence of ncAAs and their importance in determining the functional properties of both naturally occurring and synthetic peptides, the structural characterisation of peptides and proteins containing ncAAs remains challenging. For example, of the almost 200 000 structures in the Protein Data Bank (PDB), only 11 677 were annotated with a PTM (Craveur et al., 2014). This paucity of protein structures bearing ncAAs also results in them being excluded from machine learning and structure prediction algorithms, as there is an insufficient training set. Techniques used to determine the structure of proteins and peptides (X-ray diffraction, cryogenic electron microscopy and nuclear magnetic resonance (NMR)) rely heavily on modelling software to transform the experimental data into structural models (Mal et al., 2002). The amount of data that can be collected experimentally in NMR is, in general, insufficient to determine the structure of a given peptide or protein directly. Instead, a representation of the spatial arrangement of the atoms within each amino acid, together with a description of the interactions between sets of atoms, is required to translate a set of experimental restraints into a three-dimensional (3D) structure (Mal et al., 2002). Most molecular modelling packages contain only the 20 canonical amino acids and a modest selection of the most common ncAAs. This is true for both general molecular dynamics simulation packages (such as AMBER, CHARMM, GROMACS and GROMOS) as well as software dedicated to structure refinement such as Xplor-NIH (Bermejo and Schwieters, 2018), CNS (Brunger et al., 1998), ARIA (Mareuil et al., 2015; Allain et al., 2020) or CYANA (Guntert and Buchner, 2015; Guntert et al., 1997). Despite the various input formats required for the different structural calculation software packages, the internal representation of interatomic interactions is in principle the same or closely related.
Peptides containing ncAAs are ideally suited to NMR structural characterisation as they are small in size and often contain side-chain links that induce local structure (Hamada et al., 2010; Weber et al., 1991). Nevertheless, their structural characterisation by NMR is often restricted to measurements of a limited set of nuclear Overhauser effects (NOEs), hydrogen bonds or backbone chemical shifts to support specific geometries (Mendive-Tapia et al., 2015; Umstatter et al., 2020). Alternatively, NMR analysis is omitted altogether in favour of lower-resolution methods such as Circular Dichroism (CD) spectroscopy (De Araujo et al., 2022; Wu et al., 2017). The dearth of high-resolution NMR structures in this class of molecules likely stems, at least in part, from the difficulty of obtaining high-quality atomic representations of the ncAAs required for computer-assisted structure determination.
Recently, the handling of ncAAs and small molecules in CYANA was addressed by Yilmaz and Guntert (2015), who developed CYLIB, an algorithm that enables automated template generation for ncAAs and small molecules, provided that a suitable input geometry is available (CIF or Mol2 file). Here, the quality of the input geometry is critical, as the algorithm does not perform any optimisation of the structure. CYLIB has internal procedures for creating the appropriate branch structure and ring closures required by CYANA; however, practical aspects of working with ncAA-containing peptides and proteins such as consistent atom naming and side-chain linkages are not addressed by CYLIB.
For the algorithms operating in Cartesian space, topology builders have been created which take simplified representations of amino acids and other molecules as input and infer topological information based on a set of internal rules (Schmid et al., 2012; Van Der Spoel et al., 2005; Wang et al., 2006). The challenge for incorporating ncAAs lies in the use of specific atom names to infer bonded and/or non-bonded interactions, as well as assumptions regarding how individual amino acids in a peptide chain are linked or terminated. While in principle almost any molecule can be represented, users are often unaware of the assumptions that have been embedded in the code files or how to incorporate new atom types into the associated files that contain the parameter definitions needed by the builders to interpret them. Furthermore, common approaches to reduce complexity, such as using atom names to infer interaction type, work well when dealing with a small subset of chemical space (such as the 20 canonical amino acids). However, linking atom names to specific interactions rapidly leads to a combinatorial problem and an explosion in the number of terms as new classes of interactions are introduced. The addition of a single new atom type can often require the definition of hundreds of interactions to ensure compatibility with the existing framework.
In attempting to simplify the input for standard (common) cases, the authors of many programs have made the treatment of non-standard cases progressively more challenging. We have set out to address this problem and provide a generic mechanism to generate complete topological information for amino acids and related molecules of interest in a robust and reproducible manner. This can be readily translated into inputs for various simulation and structure-refinement packages. Our approach leverages the capability of the Automated Topology Builder (ATB), a publicly accessible web server that provides optimised geometries, validated force field parameters and topology files for a range of popular molecular dynamics (MD) simulation and structure-refinement packages (Stroet et al., 2018; Koziara et al., 2014; Malde et al., 2011). The ATB is provided free of charge to academic users, who can both download existing molecules and submit new structures. In the case of a new structure, the user can submit a set of Cartesian coordinates in Protein Data Bank (PDB) format together with the net charge on the molecule. Within the ATB, the geometry of the molecule is first optimised using quantum mechanical (QM) calculations. A topology is then generated by combining the results with a set of empirical rules (Malde et al., 2011). A variety of formats can be used as inputs, with the most basic being an initial set of Cartesian coordinates in Protein Data Bank (PDB) format and the net charge on the molecule.
Here, we describe an extension of the ATB that allows users to directly generate template files for a number of popular NMR structure determination software packages. A template recognition approach has been developed that recognises ncAAs (rather than “ligands”) as forming part of a peptide chain. This allows “building block” files compatible with different software packages to be generated, including 3D geometries and templates with atom names that adhere to IUPAC standards. We further introduce a general method for linking side chains of amino acids in CYANA and CNS. The utility of the procedure is demonstrated by generating models of a number of natural products containing complex ncAAs, as well as solving the structure of a side-chain cyclised synthetic peptide. We further use this method to create a publicly accessible and expanding repository of amino acids within the ATB (https://atb.uq.edu.au/index.py?tab=amino_acids, last access: 17 February 2023). The database currently holds templates for the 20 genetically encoded amino acids with different termini, common post-translationally modified amino acids, and the entire content of the SwissSidechain database (230 entries in both d- and l-form amino acids), which includes a wide range of ncAAs (Gfeller et al., 2013).
2.1 Outline
The overall workflow starts with the generation of 3D coordinates of an ncAA in a suitable amino acid template and format (described below) for submission to the ATB, where geometries are optimised and where a number of coordinate, parameter, topology and template files are generated. The CYLIB algorithm is used internally by the ATB to generate the CYANA template files from an intermediate Chemical Component Dictionary (CCD) Crystallographic Information File (CIF). All atom names are updated to adhere to IUPAC standards. The resulting outputs are made available to the user in CYANA or CNS format and stored on the ATB server (Fig. 1). Optionally, where side-chain links are required for CYANA templates, these can be generated using the CYANA Lib Linker (https://atb.uq.edu.au/cyana_linker, last access: 17 February 2023). Each component of the workflow is described in detail below.
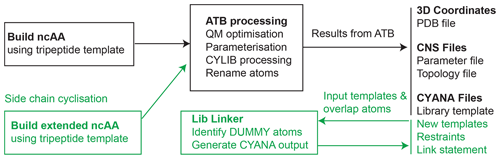
Figure 1Workflow for generating files for NMR structural calculation of peptides and proteins that contain ncAAs. The input file must be generated using the described template format (Fig. 2) and used as input to the ATB web server, which then recognises the input as an amino acid and excises the necessary portion to generate the required input files for CYANA and CNS-based structure determination software (shown in black on the right). To produce templates suitable for side-chain linkage in CYANA (shown in green), an extended ncAA template containing atoms that extend beyond the linking bond is built (Fig. 4). Two such templates are used as input to the CYANA Lib Linker interface of the ATB, where the user also defines which atoms from the first template are present in the second template and vice versa. The “overlap” atoms of the extension are changed to dummy atoms to produce a new CYANA template file (.lib file), and short upper distance restraints are generated between sets of overlapping atoms (.upl file). A link statement (to be added to the sequence file (.seq) in CYANA) is also produced to remove the repulsion between the two atoms that are to be linked.
While many pre-calculated ncAAs have been generated as described here and are stored within the ATB repository, the protocol outlined below can be followed by a user to initiate the generation of parameters (templates, topologies, etc.) for any new ncAA. New submissions of ncAAs to the ATB will be added to the existing database; thus, the repository will become progressively more complete.
2.2 Defining the group or amino acid of interest and submission to the ATB
A core feature of the protocol is how the specific group or amino acid of interest is defined and automatically recognised. To ensure an appropriate chemical environment for the parameterisation of the group of interest within a peptide chain, a series of structural templates have been defined (Fig. 2). The general form of the peptide template is Ace–Ala–X–Ala–NMe, where Ace is an N-terminal acetyl capping group, NMe is a C-terminal N-methyl amide capping group, and the central portion of the structure, denoted X, is the chemical group or amino acid of interest (Fig. 2a). Similarly, structures of the form X–Ala–NMe or Ace–Ala–X are recognised as representing an N-terminal or C-terminal residue, respectively (Fig. 2b and c). This template format allows the molecule to be identified as an amino acid and the portion “X” to be excised when generating the parameter files. Each processed entry is associated with a unique molecule ID (MOLID) within the ATB database.
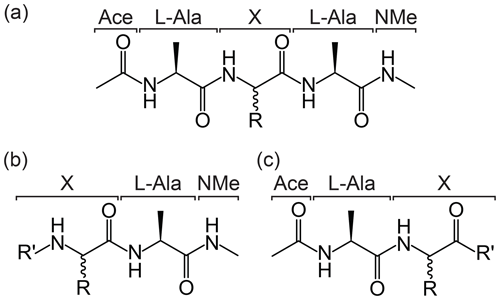
Figure 2Template formats for submission of ncAAs to the ATB. The required templates for (a) non-terminal residue, (b) N-terminal residue and (c) C-terminal residue in a peptide chain are shown. In each case, R represents the side chain of the ncAA labelled “X”, and in the case of (b) and (c) R′ represents possible modifications of the termini.
Submissions to the ATB require an unambiguous molecular representation that includes 3D coordinates (PDB format with all hydrogen atoms present) along with the net charge. There is no requirement that the input geometry is optimised; thus, it may contain clashes, non-ideal bond lengths, etc. The stereochemistry, atom information and bonded connectivities, however, must be specified correctly in the input file. The ATB submission page includes tools that will generate 3D coordinates from an embedded 2D drawing tool (JSME; Bienfait and Ertl, 2013) or a Simplified Molecular Input Line Entry System (SMILES) input. Existing entries within the ATB database can also be loaded directly into the 2D drawing tool and modified. Documentation and ATB MOLIDs for the structural templates can be found at https://github.com/ATB-UQ/CYANA-Examples (last access: 17 February 2023). There is, in principle, no limit to the size of the group of interest. However, for computational reasons, the default limit for density functional theory (DFT) calculations to be performed as part of the parameterisation by the ATB is 50 atoms, and the limit for semi-empirical QM processing is 500 atoms. Note that the most significant difference between DFT and semi-empirical QM processing by the ATB is the atomic charge model, which is not relevant for CYANA calculations as electrostatic interactions are not considered. While the group of interest is not required to be a canonical amino acid, it must be able to be incorporated into a peptide chain via peptide bonds. The procedures used by the ATB to parameterise molecules and the steps taken to validate these parameters have been described in detail elsewhere (Malde et al., 2011; Stroet et al., 2018).
All amino acid entries are listed as such on the ATB (https://atb.uq.edu.au/index.py?tab=amino_acids); this includes entries described herein, N- and C-terminal versions of all genetically encoded amino acids and all ncAAs contained within the SwissSidechain database (https://www.swisssidechain.ch, last access: 17 February 2023).
2.3 Renaming algorithm
When working with ncAAs, it is important to adhere to a consistent set of rules when naming the atoms on the side chain. Additionally, it is important for the workflow that atom names are unique. We therefore developed a tool to ensure both requirements are fulfilled. In 1969, a set of rules were recommended by IUPAC-IUBMB (1984) for the representation of proteins and peptides. A section of these rules pertains to the labelling of the constituent atoms in amino acids. These rules are adhered to in the presentation of NMR structures of proteins and peptides (Markley et al., 1998).
Non-hydrogen atoms present in the side chain of amino acids are identified based on the lowest number of bonds that separate them from the Cα atom (determined from the connectivity information in the CYANA template or Mol2 format). The order of atoms is indicated using the Greek alphabet (using corresponding Roman characters in files). The first atom connected to Cα is Cβ, Cγ is two bonds away, and so on (from position 25, the Greek alphabet is repeated as two-letter codes, i.e. Cαα, Cαβ, Cαγ until Cωω – expanding the available atoms to 600 in this method). While seemingly straightforward, complexities of this naming system arise for branched amino acid side chains, which are common in many ncAAs. In the event of a branch, where two atoms are the same number of bonds away from the backbone and therefore are assigned the same Greek letter, chain priority is assigned based on the Cahn–Ingold–Prelog (CIP) priority rules (Cahn and Ingold, 1951; IUPAC, 2013).
An algorithm was written to automatically name the side chain of amino acids (https://github.com/ATB-UQ/fixnom, last access: 17 February 2023). This involves the following steps.
- 1.
The library file containing atom coordinate and connectivity information is read as input (CYANA template format or Mol2 format). The input is parsed, and a reduced matrix is formed containing only the heavy atoms and their connectivities (to heavy atoms).
- 2.
The matrix is expanded to introduce dummy atoms to represent unsaturated bonds – these are required for the implementation of the CIP rules. The Cα atom is identified, and the matrix reordered based on this information.
- 3.
A connectivity matrix is created, describing the distance (in bonds) between any two atoms. The atoms are ordered based on their distance from the Cα atom.
- 4.
At this point, the chains are initiated by considering all atoms connected to the Cα position. All (heavy) atoms connected to Cα are given a position and a chain identifier. The position is simply the number of bonds away from Cα. The chain identifier follows the CIP rules. The chain with highest priority is assigned the lowest number, and additional chains are provided with an incremented chain number based on priority. The priority of the chain is determined as follows:
- a.
The priority of that atom (atomic number) is first.
- b.
The priority of each attached atom one bond away. If all connected atoms have the same priority, then evaluate all atoms two bonds away and so on.
- c.
If the priority cannot be resolved by the above method, the atomic coordinates of the atoms are considered, and the R/S configuration of the branching atoms are used to assign priority according to CIP rules. This last step is only required for cases of tetrahedral atoms containing two identical chains (ignoring the preceding atom in the chain). If there are three identical chains, their identity is arbitrary by rotation (and no further action is taken). Practically this is implemented by using the method of Cieplak and Wisniewski (Cieplak and Wisniewski, 2001), where the determinant of the 4×4 matrix formed by the atomic coordinates of the atoms (X, Y, Z, 1) in the stereocentre is used to evaluate if an atom is clockwise or anticlockwise in position with respect to a geminal atom. The atom with the highest priority is placed at the bottom row of the matrix, and the two top rows are then occupied by the two atoms being evaluated. As noted by Cieplak and Wisniewski, the position or the identity of the third row does not affect the handedness of the atoms being evaluated, and this atom can be “placed” at the position of the central atom. In their implementation, they do this for cases where the fourth atom is a hydrogen (often removed in databases) and show that this is valid since this atom will always have a lower priority than the other atoms. Similarly, in our case, we do not need to know what this atom is since it will always have a lower priority than the atom preceding the branch point (according to the CIP elaboration by Markley et al. (1998), the atom closest to the Cα atom always has the highest priority); thus, we can simply fill the third row with the atomic coordinates of the central atom.
- a.
- 5.
The above procedure assigns chain numbers to atoms that are one bond ahead of the atom being considered in an incremental (one-pass) approach. This requires that at each increment the atoms that have already been assigned priority (ahead of the atom being evaluated) are reordered based on their assigned priority in the previous step.
- 6.
A situation can arise where an atom is evaluated twice if it joins two branches, as is the case for rings. In these cases, the atom is given the lower chain ID between that already assigned to it and what would be assigned to it had it not been a joining atom.
Once all the chains have been generated, a procedure for re-evaluating identical chains (due to symmetry) in different parts of the molecule is applied. Here the chain number is reordered based on the priority of the chains from which they branch. This is not explicitly defined by the CIP rules but is required to ensure that if a branch contains two identical aromatic rings the chain numbers in each aromatic ring are consecutive (i.e. to avoid a ring having chain identifiers 1 and 3 or 2 and 4).
Once all chains have been created, the chain number and the distance from the Cα atom are used to generate the correct atom names. Note that if a position exists that belongs to chain 1 and only one (heavy) atom occupies this position (number of bonds away from Cα), then this atom is not given a chain number, and only the Greek alphabet character corresponding to the bond position is used. Finally, hydrogen atoms (and pseudoatoms) are added. For cases where two hydrogens are attached to a tetrahedral carbon, the stereochemistry of these is used to assign priority (using the same method as outlined above in step 4c).
This algorithm has been implemented in the ATB using an open-source standalone Perl script developed in-house (https://github.com/ATB-UQ/fixnom) and is used to apply IUPAC atom naming to all amino acid building-block outputs. This algorithm is used internally in the ATB to rename atoms in all output files, including PDB files that can be used to define atomic templates in NMR analysis software such as CCPN (available in v2 and planned for v3; Skinner et al., 2016; Vranken et al., 2005). An example of a complex ncAA named by the algorithm is shown in Fig. 3.
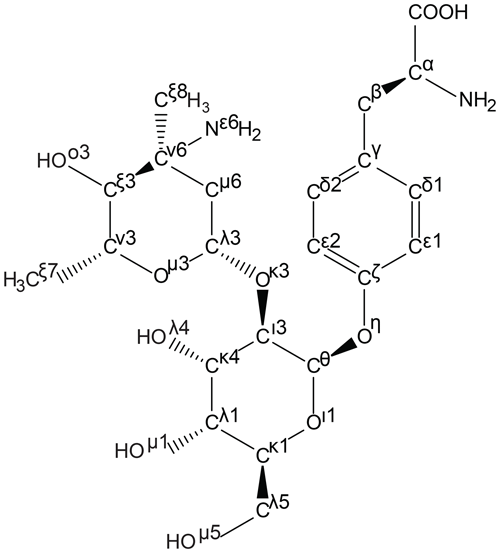
Figure 3Generation of template files following the IUPAC atom-naming conventions for amino acids. As an example, the atom names of the ncAA d-phenyl-Gly* (ATB MOLID 606467), present in vancomycin, were automatically generated using an in-house algorithm. Greek letters are used to signify the number of bonds between a given atom and the Cα atom, with β denoting 1 bond, γ denoting 2 bonds and continuing to o denoting 14 bonds away. Chain priority is shown by the number next to the Greek letter. In this diagram several important priority assignment examples can be seen. For example, at the branch of Cθ to Cι and Oι, priority is assigned to the chain with the heavier atom, oxygen; hence, the priorities reflect Cι3 and Oι1.
2.4 ATB–CYLIB–CYANA pipeline
Due to the torsion angle dynamics algorithm used by CYANA to efficiently sample configurations, the parameters used to describe amino acids are arranged according to a tree structure with the N terminus at the base and the side chains (and C terminus) as terminating branches (Guntert and Buchner, 2015). Because of the inherent complexities in representing molecules in this manner, the ATB utilises the CYLIB application to produce a CYANA library file for amino acid building blocks (Yilmaz and Guntert, 2015). This is achieved by excising the target group (X) from the templates outlined in Fig. 2 and producing a CIF containing the variables and amino acid backbone atoms (and atom names) to match amino acid structures within the CCD (Westbrook et al., 2015). The resulting CCD-compatible amino acid CIF is passed to CYLIB with the arguments required for side chain, C- or N-terminus groups, and the resulting files are made available as downloadable files on the ATB site. Note that in cases where side chains or termini contain cyclic elements (within an amino acid), CYLIB also produces a restraint macro to close the cycle, which is also provided as a downloadable file.
2.5 CYANA Lib Linker distance restraints
To enable amino acid side-chain and backbone cyclisation within CYANA, the ATB provides a tool to generate distance restraints, linking statements and modified library files called CYANA Lib Linker (https://atb.uq.edu.au/cyana_linker).
The CYANA Lib Linker takes two input CYANA library files both of which contain the two atoms that will form the link between the two amino acids plus at least a one atom extension beyond the linking bond (e.g. a disulfide bridge could be formed by linking two side chains of cysteines in the form Cα–Cβ–Sγ–Sγ'–Cβ') – see Fig. 4. To make the process generic for any linkage, templates are required for each side of the link (in the disulfide bond example the same template is used twice as input). For each template the following must then be defined: (1) “Residue index”, which is the residue number in the intended peptide sequence; (2) “Linking bond”, defined by the two atoms involved in the side-chain linkage (Sγ–Sγ'); (3) “overlap atoms” defined as atoms that exist in both templates (these atoms may have different names – in the disulfide bond example, this would be a process of pairing atoms Cβ of one template with the Cβ' of the other and Sγ of one with the Sγ' of the other). Once all of the above is satisfied, the algorithm edits the input template files by altering the atom type of the overlap atoms (and attached hydrogens) in the library extension (Sγ'–Cβ') as “DUMMY” (excluding “PSEUDO” atoms which are not altered). An upper distance is defined for each pair of corresponding overlap atoms with a limit of 0.04 Å (chosen arbitrarily, must be larger than 0 and within experimental uncertainty) and assigned a weight of 10 (i.e. equivalent to 10 NOEs in CYANA). CYANA Lib Linker also produces a link statement that removes the repulsion term between the bound atoms (between the two Sγ atoms from each template) within CYANA. The link statement is included in the sequence file input of CYANA.
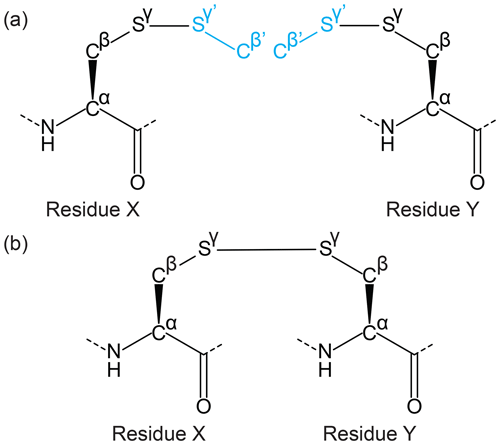
Figure 4Example of a side-chain cyclisation using the Lib Linker for CYANA. Two extended ncAA template files are generated representing either side of the linkage (extension shown in cyan), including the linking atom and neighbouring atoms that define the chemical environment of the linked atoms. (a) For a disulfide bond, the linked residues are the same on either side, and a single template can be selected as both input files for Lib Linker (residues X and Y). Each side is, however, defined as having different positions in the peptide chain. The connecting atoms (Sγ from each template) and overlap atoms (Cβ and Sγ overlap with Cβ' and Sγ' of the other template, shown in black and cyan respectively) are provided as user-defined input to Lib Linker. (b) Lib Linker generates two templates (identical in this case), where only the atoms from each side of the linking bond are included. Restraint files are also generated that can be used directly for CYANA structure calculations.
2.6 ATB–CNS pipeline
MD force fields have been incorporated into several NMR structure determination packages including XPLOR (currently distributed as Xplor-NIH), ARIA and CNS (Schwieters et al., 2003, 2006; Bermejo and Schwieters, 2018). For non-backbone-modified ncAAs lacking side-chain cyclisation, implementation into CNS force fields for structure calculations is straightforward. The ncAA is built and processed by the ATB as described above. To maintain compatibility with the current protein force field used by CNS, the amino acid building-block files produced by the ATB retain the standard CNS atom types as well as the bonded and non-bonded parameters for atoms involving protein backbone definitions (N, HN, CA, HA, CB, O). This allows standard linkage statements to be used for producing the molecular template. The new topology and parameters can simply be added to the standard files without modification. New atom types are defined for all other atoms in the ncAA, allowing the new geometry and charges to be defined. In order to conserve the net charge when combining the CNS protein backbone charges with the ATB charge model, any residual charge is simply added to the non-backbone atom with the largest magnitude charge. Note that while the duplication of atom types is prevented between ATB outputs and the standard CNS protein force field, atom types defined within separate ATB outputs are not unique. In cases where multiple and distinct ATB parameterised groups are being used within a single peptide, manual resolution of atom types to ensure they are unique may be required.
For side-chain-linked ncAAs, a manual addition of the cross-link is required. As for the CYANA approach, generating building blocks with overlapping atoms allows for all aspects of the required geometry to be defined and for topology and parameters to be directly added to the existing force field. A specific linking statement that removes the extra atoms; modifies charges if required; and adds the required bonds, angles, and improper dihedrals (analogous to how disulfide bonds are implemented) can subsequently be written by the user. Similarly, ncAAs that include backbone modifications (and thus cannot be modelled using the existing standard linkage statements for creating peptide bonds) also require manual modification of the peptide bond linkage statement to be incorporated and modelled correctly.
3.1 Disulfide bonds
The handling of side-chain linkages described here is a departure from the default approach used in CYANA for linking disulfide bonds. Currently, disulfide bonds are defined using a special template file called CYSS, where the template contains all atoms up to the linking sulfur atom. A set of upper and lower one- and two-bond distance limits are then imposed to maintain an appropriate geometry around the introduced bond. One problem with this approach is that the χ2 and χ3 torsion angles of disulfide bonds are not defined in the template and are therefore neither explicitly subject to the CYANA torsion angle search nor can they be easily constrained to specific values.
To validate the use of the new template (CYSX), we recalculated the structures of a number of disulfide-rich peptides resolved in our group (e.g. 2KSL, 5LIC) using the new template (data not shown). The recalculated structures show no clear differences to those previously calculated using the CYSS template, and distance restraints to define the disulfide bond (average χ2 and χ3 torsion angles in disulfide bonds, obtained using either method) are within the spread of the equivalent angles generated for each method. We note that using the CYSX template instead of the CYSS template in CYANA requires only trivial changes to the associated files. Specifically, (1) CYSS is replaced with CYSX in the sequence file (the “link” statement which removes the repulsion between the connected sulfur atoms is used in both approaches and requires no further changes); (2) CYSX is appended to the existing CYANA library; (3) the old restraints for upper and lower distance limits to define the disulfide bond are removed and replaced with the new short upper distance limits between “overlap atoms”; (4) after structure calculation the DUMMY atoms are removed, and the CYSX name changed to CYS. The last point can be achieved by adding four lines of commands to the end of the CYANA structure calculation script (using existing CYANA functions). Warnings may occur due to a conflict between CYSX and CYSS in other restraints files (i.e. dihedral angle restraints), but these can be ignored. The required template file for CYSX, the associated distance restraints and CYANA commands for removing the DUMMY atoms and producing a PDB file suitable for subsequent analysis and deposition to the PDB are provided in our GitHub repository (https://github.com/ATB-UQ, last access: 17 February 2023).
3.2 ncAAs in complex natural products
To demonstrate the capability and workflow, we have selected three examples of ncAA-containing peptides and generated the additional library files required for structure calculation by CYANA. These peptides were selected to demonstrate particular aspects of our pipeline and highlight potential practical applications. For each example, ncAA templates and restraints were generated following the above procedure, and we demonstrate that these lead to chemically sound structures during unrestrained structure calculation in CYANA.
Tyrocidine is a cyclic decapeptide antibiotic (Loll et al., 2014) (Fig. 5a), and it contains one ncAA which is currently not present in the standard CYANA library. The missing ornithine (at position 9) library file was generated by building the tripeptide Ace–Ala–Orn–Ala–NMe in PyMOL (using the PyMOL Builder), and the resulting PDB file was saved and submitted to the ATB (MOLID 467880). The resulting ATB entry provides all necessary files for NMR structure calculation. The CYANA template generated by the ATB was appended to the CYANA library. Existing procedures in CYANA were used to create a backbone linkage to cyclise the peptide chain. Unrestrained CYANA calculations were performed, resulting in an ensemble of chemically feasible structures (without NMR restraints). An example structure is shown in Fig. 5b.
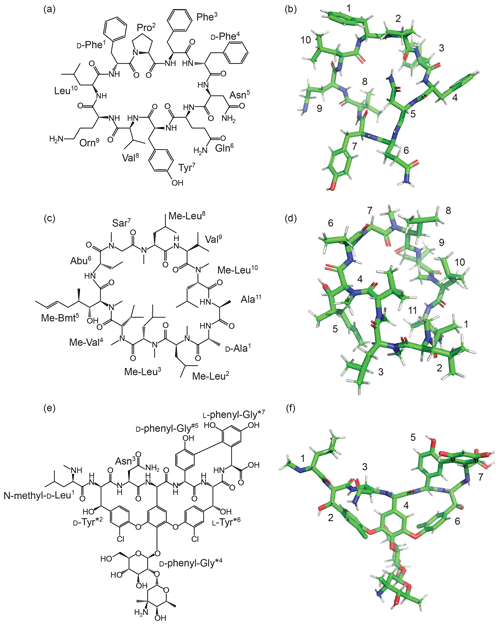
Figure 5Structures of natural products generated from un-restrained CYANA calculations using ncAA templates generated by the ATB as described herein. (a) 2D and (b) 3D structures of tyrocidine containing a single ncAA (ornithine). (c) 2D and (d) 3D structures of cyclosporine. The sequence of cyclosporine contains five ncAAs: N-methyl-leucine (Me-Leu), N-methyl-valine (Me-Val), (4R)-4-[(E)-2-butenyl]-4,N-dimethyl-l-threonine (Me-Bmt), α-aminobutyric acid (Abu), and sarcosine (Sar). (e) 2D and (f) 3D structures of vancomycin. The amino acids of vancomycin have been abbreviated in some cases. d-Tyr*2 is 3-chloro-β-hydroxy-d-Tyr; d-phenyl-Gly*4 is (2-[α-4-l-epi-vancosaminyl]-β-1-d-glucosyl)-d-phenyl-Gly; d-phenyl-Gly#5 is 4-hydroxy-d-phenyl-Gly; l-Tyr*6 is 3-chloro-β-hydroxy-l-Tyr; and l-phenyl-Gly*7 is 3,5,-dihydroxy-l-phenyl-Gly. The 3D structures were generated by CYANA based on the templates obtained by the ATB–CYLIB–CYANA pipeline without experimental restraints.
Cyclosporine (Corbett et al., 2021) is an 11-residue backbone-cyclised peptide commonly used as an immunosuppressant to treat rheumatoid arthritis and Crohn's disease, as well as in the prevention of organ rejection in transplants. Cyclosporin contains six ncAAs (Fig. 5c), five of which are not present in the standard CYANA library. The ncAAs are as follows: d-alanine (residue 1), (4R)-4-[(E)-2-butenyl]-4,N-dimethyl-l-threonine (Me-Bmt) (residue 5), α-aminobutyric acid (Abu) (residue 6) and three N-methylated amino acids (sarcosine, residue 7; N-methyl-valine, residue 4; N-methyl leucine, residues 2, 3, 8 and 10). Overall, cyclosporine required five new library files to be defined and appended to the CYANA library (d-alanine can be generated from alanine using the “library mirror” command in CYANA). A tripeptide template was generated for each and submitted to the ATB. The ncAAs needed to describe cyclosporine are now available with the following MOLIDs: N-methyl leucine, 1175924; N-methyl-valine, 1175930; Me-Bmt, 1175933; Abu, 1175938; and sarcosine, 1175941. Feasible structures were again obtained following unrestrained torsion angle dynamics simulations using CYANA (Fig. 5d).
The final example, vancomycin, is a glycopeptide antibiotic that has been included on the WHO list of most essential medicines (Schafer et al., 1996). It was, until recently, used as an antibiotic of last resort. This tricyclic heptapeptide is an example of a non-ribosomal peptide. Five of the seven amino acids in vancomycin are involved in side-chain–side-chain branches. These residues must be generated individually and subsequently processed through the CYANA Lib Linker algorithm to define overlap atoms and distance restraint files. Additional distance restraint files are also required to close the sugar rings present in residue 4.
Three side-chain links are present in vancomycin, the first (link 1) between residues 2 and 4, the second (link 2) between residues 4 and 6, and the third (link 3) between residues 5 and 7. Templates were generated with extensions beyond the linking atom (including the aromatic rings on either side of the ether bond – MOLIDs: 1212202 (residue 2) and 1212203 (residue 4) for link 1. The overlap atoms included the bonding atom (Oη3 and Cδ1 in link 1 and Cδ2 and Oη3 for link 2), as well as all atoms one bond away from the linking atom (Cζ3, Cι3 and Cι4 in residue 2 and Cζ1, Cε3 and Cγ1 in residue 4). For link 2, one additional extended library file (MOLID: 1213034) was generated for residue 6, and the following overlap atom mapping was used: Cγ2, Cε3 and Cζ2 in residue 4 and Cι4, Cι3 and Cζ3 in residue 6. Note that the modified residue 4 from link 1 is used as input when creating link 2. For link 3, between residues 5 and 7, two additional library files were generated, MOLIDs 1212698 and 1212205, respectively. Link 3 exists between the bonding atoms Cδ1 and Cγ3 with overlap atom mapping: Cγ1, Cε1, Cζ3 and Cζ4 in residue 5 and Cε5, Cε3, Cδ1 and Cβ in residue 7. Finally, a template was produced for the N-terminal residue (MOLID 1212206). Using these template files, unrestricted torsion angle dynamics were performed in CYANA (Fig. 5f).
Vancomycin contains two sugar rings, and in CYANA each must be “closed” using an internal ring-closure process. CYLIB automatically generates the required CYANA commands for closing rings; however, it currently cannot handle multiple ring closures, such as those for residue 4 of vancomycin. This required an additional ring-closure statement to be added manually. An additional manual step involved adding a torsion angle that defines the angle between the two aromatic rings that are directly fused (between residues 5 and 7).
3.3 Practical application using an engineered peptide
Advances in peptide chemistry are rapidly expanding the chemical space accessible to high-throughput peptide synthesis methods. Recently, a systematic approach was taken to explore side-chain stabilisation of a segment of the amyloid precursor protein (APP; 1NGYENPTYKFFE12) into the conformation it adopts when bound to the phosphotyrosine binding (PTB) domain of Mint2 (Bartling et al., 2023). The method described herein was developed to solve the structure of four peptides with different side-chain linkages (Bartling et al., 2023). One of these peptides is side-chain-cyclised through ring-closing metathesis (RCM) between residues 7 and 11. The 3D structure of this peptide has been reported elsewhere (Bartling et al., in review) and was solved using the methods described herein. The side-chain cyclisation following RCM was, however, treated using a similar approach to that currently used in CYANA for fusing sulfur atoms in disulfide bonds (i.e. using truncated template files). Here, we revisit this structure and perform the side-chain fusion using the new approach described here. We further demonstrate how CNS templates, generated by the ATB, can be used for water refinement of the reported CYANA structure.
First, Thr7 and Phe11 were replaced with the α-methyl-substituted olefin-bearing ncAA named pentenyl alanine “PAL” (MOLID 929126). This is an extended form of the PAL side chain as shown in Fig. 6. The CYANA Lib Linker was then used to modify the template (DUMMY and overlap atom definition) and to generate appropriate upper distance restraints. For the terminal residues, templates corresponding to the N-terminal Asn and C-terminal Glu (MOLIDs 1162954 and 1159438) with their respective amino and carboxy acid termini were also generated.
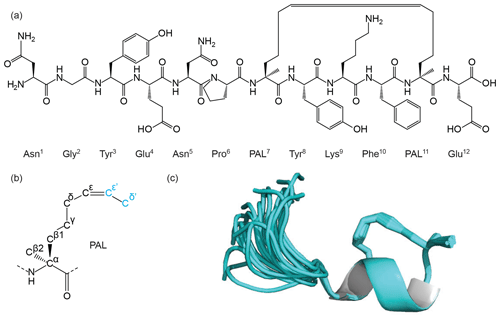
Figure 6Figure showing the chemical structure of a synthetic peptide (a) that mimics the Mint-2 bound form of the amyloid precursor protein (APP). The helical structure in the C-terminal tail of the peptide is stabilised through side-chain cyclisation using ring-closing metathesis (RCM). The RCM linkage was modelled using an extended pentenyl alanine (PAL) library entry (b) generated by the ATB. The ATB-generated library was further processed by the CYANA Lib Linker interface of the ATB to generate a new template where the two overlap atoms, Cε' and Cδ', are converted to DUMMY atoms and used to constrain the side chain around the double bond. The 3D structure of the peptide was determined using CYANA and then further refined in explicit water using CNS (c) with topology and parameter files generated by the ATB. The structure shows stabilisation of the helical motif (cartoon), and the PAL side chain is shown as sticks.
To assign the magnetic resonances of the peptide, CcpNmr Analysis 2.4.1 was employed (Vranken et al., 2005). The atomic composition of individual ncAAs in CcpNmr was defined by uploading the ATB-generated coordinate files to its molecule library. Resonance assignments were obtained using a combination of 2D 1H–1H TOCSY, 2D 1H–1H NOESY, and natural abundance 2D 1H–15N and 1H–13C HSQC. The 1H chemical shifts were calibrated with reference to the water chemical shift, while 13C and 15N chemical shifts were calibrated indirectly with reference to 1H. Cross-peaks from the 2D 1H–1H NOESY (mixing time of 350 ms) were manually picked to generate a list of interproton distance restraints. TALOS-N (Shen and Bax, 2013) was used to derive dihedral angle restraints. As TALOS-N does not recognise ncAAs, we replaced the ATB-generated residue codes for the terminal residues with N and E, respectively, in the TALOS-N input file. The angle restraint range was set to twice the estimated standard deviation.
To perform calculations using CYANA, the following files were prepared using ATB, CcpNmr and TALOS-N: (i) a sequence file listing the amino acid sequence of the peptide, (ii) a chemical shift file listing the chemical shifts of all assigned atoms, (iii) a peak list of the 2D 1H–1H NOESY spectrum containing the chemical shifts and calibrated peak intensity (height or volume) of each peak, (iv) an angle restraint file derived from TALOS-N, (v) a CYANA library file specific for the ncAA templates acquired from the ATB, and (vi) restraint files specific for the side-chain linkage generated by CYANA Lib Linker. The sequence file and peak list (distance restraints) were directly exported from CcpNmr using the CYANA-compatible XEASY format. The chemical shifts of protons in the peptide were first exported from CcpNmr in BMRB format (any other formats would omit the entries for any ncAAs – a current CcpNmr limitation). The shift list in BMRB format was imported into CYANA, and pseudoatoms were added using an internal CYANA command. CYANA (v. 3.98.13) was then used to automatically assign the peak list, extract distance restraints and calculate 200 structures from which 20 structures with lowest target function values were selected to represent the structure ensemble of the peptide.
The output of the CYANA structure calculation was used to perform water refinement in CNS. The CNS topology and parameter output for PAL was added to the standard force field, and this residue was included at both positions to be linked. A linkage statement deleting the extra atoms was subsequently introduced to generate a complete molecular template file with all the atoms and restraints required to define the geometry of the residue. Because of the additional backbone methyl group, a custom peptide bond linkage statement was also constructed and used to create the residue links on either side of each ncAA (both custom linkage statements are also available at https://github.com/ATB-UQ). Structures were calculated and minimised in water using the experimental distance and angle restraints, resulting in a well-defined family of structures with excellent geometry and no violations of the experimental data (Fig. 6c and Table 1).
A workflow has been developed that facilitates the structure determination and refinement of peptides and proteins that contain ncAAs using popular NMR software, including CYANA and CNS. The methodology has been incorporated into the ATB server, which allows arbitrary ncAAs to be built as required. New ncAAs are stored on the site and added to a database of entries containing all of the necessary files for structure determination by NMR spectroscopy. The workflow introduces a number of automated procedures to improve access to complex ncAAs without manual intervention. An algorithm has also been developed that can automatically assign IUPAC atom names to ncAAs. It has already been highlighted that there have been errors within the PDB when naming for diastereotopic atoms (Bottoms and Xu, 2008). The potential explosion in elaborate side chains that is possible when introducing ncAAs requires that care is taken when defining atoms, reporting and comparing data.
We have also introduced into this workflow a standalone and general method for introducing side-chain-to-side-chain bonds in CYANA. A graphical user interface has been incorporated into the ATB to facilitate this process using user-supplied template files (i.e. the files generated within the server). The new side-chain linking procedures use overlap “DUMMY” atoms to establish the missing bond in CYANA. This is a departure from the standard method currently used in CYANA for linking side-chain atoms of pairs of cysteines. Tests of this procedure showed that the structures produced by both the new and old methods were very similar. Importantly, the new approach allows for definition of the χ2 and χ3 torsion angles in cysteine bridges, enabling these to be sampled by the torsion angle dynamics in CYANA, and for these angles to be defined where experimental evidence is available (Armstrong et al., 2018; Ramanujam et al., 2019). In the traditional approach of defining disulfide bonds, these torsion angles are not defined, and disulfide bond geometries are instead sampled indirectly by altering the χ1 angles and the consequences of this on the imposed distance restraints and repulsion terms associated with the other side-chain atoms. While the result appears to be similar using the two methods in the absence of χ2 and χ3 restraints, the new method will certainly be favourable where such data are available. More generally, this procedure will allow all side-chain links to include definition of inter-residue torsion angles in CYANA.
The utility of the workflow was demonstrated by building structural models of several natural products in CYANA. In most cases only a single ncAA is present in a peptide or protein chain, due to incorporation of the ncAA via recombinant expression methods, via peptide synthesis or via chemical modification of specific amino acids in the produced peptide or protein. This was captured by modelling tyrocidine, a short peptide incorporating a single ncAA. In such cases, the method is very robust, and little manual intervention is required (beyond adding the additional templates to the CYANA library). Cases involving multiple ncAAs, especially those containing backbone N-methylation, are very challenging to prepare manually; however, even cyclosporin poses no issues in our pipeline beyond the once-off generation of the template files. The most challenging example explored, the antibiotic vancomycin, is an extreme case involving multiple interlocked ring structures formed by linking ncAAs. CYLIB currently cannot automatically process groups with multiple rings, such as residue 4 in vancomycin which has two sugar moieties branching from an aromatic ring. Thus, some manual steps are required to add the extra ring-closure statements. Similarly, the central residue 4 of vancomycin is linked by a side chain to two different residues. This requires serial iterations of the CYANA Lib Linker interface to generate the required files – i.e. first templates for residues 2 and 4 are used to generate modified templates and then the modified template for residue 4 is further modified when submitted as the linking residue to residue 6. Even this challenging molecule could be processed using the tools developed.
The results described above demonstrate that the ncAA templates are compatible with existing libraries and yield high-quality structures. We also showed how one might use the templates in conjunction with experimental data. The APP-derived peptide that includes an RCM-cyclised side chain was used to highlight some practical considerations associated with the pipeline described in this work. Some manual processing is still required to use our templates with the popular CcpNmr software. While we were able to create a working solution for v2 of CcpNmr, incorporation of ncAAs within a peptide chain in v3 is currently not feasible. Other analysis packages such as POKY do not require templates and may be more suited for use with ncAAs (Lee et al., 2021). We also noted that additional automation was required to appropriately import the output of CcpNmr into CYANA. Although we have a working solution, it is likely that future development of CcpNmr will address these problems that exist when working with ncAAs.
The final test involved the refinement of the APP peptide in water using the CNS software. This currently involves manual creation of the linkage statements. This is relatively straightforward, involving a simple modification of the standard peptide bond and/or the standard disulfide bond definitions. Importantly, because the geometries are already defined in the building blocks and because all parameters required are included in the ATB output, the statement simply has to define which atoms are linked and infer other geometrical constraints such as the planarity of the double bond. Once written, these linkage statements can be used for any variant of a given ncAA. For example, the peptide backbone link used for the PAL residue can be applied to any residue type in which the Hα proton has been replaced with a methyl group.
The manual modifications that were noted in specific cases are largely due to limitations and compatibility issues of the tools used in the pipeline. The treatment of ring closures by CYLIB has been noted previously. The need for a torsion angle between connected aromatic rings is a consequence of existing rules within CYLIB. The limitations within the import function of CcpNmr is subject to current development by the authors of that software. The manual steps required for compatibility with CNS are largely due to the use of atom types to define both Lennard–Jones parameters and the bonded terms. Name clashes in the atom type definitions that arise from combining multiple ATB-generated building blocks within a single system must be addressed to ensure the intended parameters are used. Further refinement of the ATB outputs to improve compatibility with different packages (e.g. NIH-Xplore, AMBER, ARIA) will be the subject of future work.
The workflow presented here was developed to cater to the rapid growth in peptide and protein engineering in recent years, with examples including directed evolution mRNA display methods to generate macrocyclic peptide ligands of target receptors (Goto and Suga, 2021), modified amino acids for structural studies (Mekkattu Tharayil et al., 2022) and high-throughput chemical synthesis in drug development (Bartling et al., 2023). These developments have increased interest in solving structures of ncAA-containing peptides, leading us to develop the described method. The protocols and tools we have developed are designed to be general and to interface with a range of software packages. That said, it is likely that not all ncAAs that can be envisaged will be able to be handled with the existing workflow. Nevertheless, the solution we presented in this work not only addresses a pressing need in the cases of ncAAs but also provides a general framework that can be used to improve the description of amino acids in structure refinement more broadly.
Peptides containing ncAAs encompass a large pool of biologically active molecules with many potential industrial, agricultural and pharmaceutical uses. The significant structural diversity found in these peptides presents significant challenges for NMR spectroscopists when applying existing structure determination tools. This problem requires the development of a set of tools that can automatically generate molecular representations that have suitable chemical properties. We have here provided a solution to this problem in the form of an extension to the Automated Topology Builder, which can now produce template files compatible with most commonly used NMR structure calculation software. We have also ensured that the ncAA templates generated adhere to IUPAC naming conventions based on the Cahn–Ingold–Prelog priority rules. This extends the utility of NMR structure calculation to complex natural products; synthetic peptides; and complex, natural and unnatural, post-translational modifications.
The code used in this work is publicly available on GitHub and can be accessed via the following DOIs: https://doi.org/10.5281/zenodo.7655274 (Mobli et al., 2023), https://doi.org/10.5281/zenodo.7655279 (Kuschert and Stroet, 2023), https://doi.org/10.5281/zenodo.7649886 (Mobli and Stroet, 2023), and https://doi.org/10.5281/zenodo.7649851 (Rosengren and Stroet, 2023). All of the experimental data used are from previously published studies which are cited.
SK, MS, MM and AEM conceived of the project and designed elements of the pipeline. MS implemented the code in the ATB with input from AEM. SK validated the implementation with input from MM. MS, TL and MM wrote code used in the ATB workflow. SK, YKYC, ACC, XJ, KJR and MM produced the models presented and analysed the NMR data. CROB and KS provided the synthetic APP peptide. PG contributed to CYANA template generation and the side-chain linking method. SK wrote the first draft of the manuscript, and all authors contributed to the final version.
The contact author has declared that none of the authors has any competing interests.
Publisher's note: Copernicus Publications remains neutral with regard to jurisdictional claims in published maps and institutional affiliations.
This project was supported by The University of Queensland (Postgraduate Research Scholarship to Sarah Kuschert, Research Stimulus fellowship to Yanni Ka-Yan Chin and Development Fellowship to Mehdi Mobli).
This research has been supported by the Australian Research Council (grant nos. DP190101177, DP220103028, and DP220100896), the Australian National Health and Medical Research Council (grant no. APP1162597 to Mehdi Mobli), and the Austrian Science Fund (FWF) (grant no. P36101-B to Anne Claire Conibear).
This paper was edited by Nicola Salvi and reviewed by Bruno Kieffer and two anonymous referees.
Aebersold, R., Agar, J. N., Amster, I. J., Baker, M. S., Bertozzi, C. R., Boja, E. S., Costello, C. E., Cravatt, B. F., Fenselau, C., Garcia, B. A., Ge, Y., Gunawardena, J., Hendrickson, R. C., Hergenrother, P. J., Huber, C. G., Ivanov, A. R., Jensen, O. N., Jewett, M. C., Kelleher, N. L., Kiessling, L. L., Krogan, N. J., Larsen, M. R., Loo, J. A., Ogorzalek Loo, R. R., Lundberg, E., MacCoss, M. J., Mallick, P., Mootha, V. K., Mrksich, M., Muir, T. W., Patrie, S. M., Pesavento, J. J., Pitteri, S. J., Rodriguez, H., Saghatelian, A., Sandoval, W., Schluter, H., Sechi, S., Slavoff, S. A., Smith, L. M., Snyder, M. P., Thomas, P. M., Uhlen, M., Van Eyk, J. E., Vidal, M., Walt, D. R., White, F. M., Williams, E. R., Wohlschlager, T., Wysocki, V. H., Yates, N. A., Young, N. L., and Zhang, B.: How many human proteoforms are there?, Nat. Chem. Biol., 14, 206–214, https://doi.org/10.1038/nchembio.2576, 2018.
Allain, F., Mareuil, F., Menager, H., Nilges, M., and Bardiaux, B.: ARIAweb: a server for automated NMR structure calculation, Nucleic Acids Res., 48, W41–W47, https://doi.org/10.1093/nar/gkaa362, 2020.
Armstrong, D. A., Kaas, Q., and Rosengren, K. J.: Prediction of disulfide dihedral angles using chemical shifts, Chem. Sci., 9, 6548–6556, https://doi.org/10.1039/c8sc01423j, 2018.
Barber, K. W. and Rinehart, J.: The ABCs of PTMs, Nat. Chem. Biol., 14, 188–192, https://doi.org/10.1038/nchembio.2572, 2018.
Bartling, C. R. O., Alexopoulou, F., Kuschert, S., Chin, Y. K. Y., Jia, X., Sereikaite, V., Özcelik, D., Jensen, T. M., Jain, P., Nygaard, M. M., Harpsøe, K., Gloriam, D. E., Mobli, M., and Strømgaard, K.: Comprehensive Peptide Cyclization Examination Yields Optimized APP Scaffolds with Improved Affinity toward Mint2, J. Med. Chem., https://doi.org/10.1021/acs.jmedchem.2c02017, 2023.
Bermejo, G. A. and Schwieters, C. D.: Protein Structure Elucidation from NMR Data with the Program Xplor-NIH, Methods Mol. Biol., 1688, 311–340, https://doi.org/10.1007/978-1-4939-7386-6_14, 2018.
Bienfait, B. and Ertl, P.: JSME: a free molecule editor in JavaScript, J. Cheminformatics, 5, 24, https://doi.org/10.1186/1758-2946-5-24, 2013.
Bondalapati, S., Jbara, M., and Brik, A.: Expanding the chemical toolbox for the synthesis of large and uniquely modified proteins, Nat. Chem., 8, 407–418, https://doi.org/10.1038/nchem.2476, 2016.
Bottoms, C. A. and Xu, D.: Wanted: unique names for unique atom positions. PDB-wide analysis of diastereotopic atom names of small molecules containing diphosphate, BMC Bioinformatics, 9, S16, https://doi.org/10.1186/1471-2105-9-S9-S16, 2008.
Brunger, A. T., Adams, P. D., Clore, G. M., DeLano, W. L., Gros, P., Grosse-Kunstleve, R. W., Jiang, J. S., Kuszewski, J., Nilges, M., Pannu, N. S., Read, R. J., Rice, L. M., Simonson, T., and Warren, G. L.: Crystallography & NMR system: A new software suite for macromolecular structure determination, Acta Crystallogr. D, 54, 905–921, https://doi.org/10.1107/s0907444998003254, 1998.
Bullwinkle, T., Lazazzera, B., and Ibba, M.: Quality control and infiltration of translation by amino acids outside of the genetic code, Annu. Rev. Genet., 48, 149–166, https://doi.org/10.1146/annurev-genet-120213-092101, 2014.
Caboche, S., Pupin, M., Leclere, V., Fontaine, A., Jacques, P., and Kucherov, G.: NORINE: a database of nonribosomal peptides, Nucleic Acids Res., 36, D326–331, https://doi.org/10.1093/nar/gkm792, 2008.
Cahn, R. S. and Ingold, C. K.: 131. Specification of configuration about quadricovalent asymmetric atoms, J. Chem. Soc., 612–622, https://doi.org/10.1039/jr9510000612, 1951.
Chuh, K. N., Batt, A. R., and Pratt, M. R.: Chemical Methods for Encoding and Decoding of Posttranslational Modifications, Cell Chem. Biol., 23, 86–107, https://doi.org/10.1016/j.chembiol.2015.11.006, 2016.
Cieplak, T. and Wisniewski, J. L.: New Effective Algorithm for the Unambiguous Identification of the Stereochemical Characteristics of Compounds During Their Registration in Databases, Molecules, 6, 915–926, https://doi.org/10.3390/61100915, 2001.
Coin, I.: Application of non-canonical crosslinking amino acids to study protein-protein interactions in live cells, Curr. Opin. Chem. Biol., 46, 156–163, https://doi.org/10.1016/j.cbpa.2018.07.019, 2018.
Conibear, A. C.: Deciphering protein post-translational modifications using chemical biology tools, Nature Reviews Chemistry, 4, 674–695, https://doi.org/10.1038/s41570-020-00223-8, 2020.
Corbett, K. M., Ford, L., Warren, D. B., Pouton, C. W., and Chalmers, D. K.: Cyclosporin Structure and Permeability: From A to Z and Beyond, J. Med. Chem., 64, 13131–13151, https://doi.org/10.1021/acs.jmedchem.1c00580, 2021.
Craveur, P., Rebehmed, J., and de Brevern, A. G.: PTM-SD: a database of structurally resolved and annotated posttranslational modifications in proteins, Database-Oxford, 2014, bau041, https://doi.org/10.1093/database/bau041, 2014.
de Araujo, A. D., Lim, J., Wu, K. C., Hoang, H. N., Nguyen, H. T., and Fairlie, D. P.: Landscaping macrocyclic peptides: stapling hDM2-binding peptides for helicity, protein affinity, proteolytic stability and cell uptake, RSC Chem. Biol., 3, 895–904, https://doi.org/10.1039/d1cb00231g, 2022.
Gfeller, D., Michielin, O., and Zoete, V.: SwissSidechain: a molecular and structural database of non-natural sidechains, Nucleic Acids Res., 41, D327–332, https://doi.org/10.1093/nar/gks991, 2013.
Goodrich, A. C. and Frueh, D. P.: A nuclear magnetic resonance method for probing molecular influences of substrate loading in nonribosomal peptide synthetase carrier proteins, Biochemistry, 54, 1154–1156, https://doi.org/10.1021/bi501433r, 2015.
Goto, Y. and Suga, H.: The RaPID Platform for the Discovery of Pseudo-Natural Macrocyclic Peptides, Accounts Chem. Res., 54, 3604–3617, https://doi.org/10.1021/acs.accounts.1c00391, 2021.
Guntert, P. and Buchner, L.: Combined automated NOE assignment and structure calculation with CYANA, J. Biomol. NMR, 62, 453–471, https://doi.org/10.1007/s10858-015-9924-9, 2015.
Guntert, P., Mumenthaler, C., and Wuthrich, K.: Torsion angle dynamics for NMR structure calculation with the new program DYANA, J. Mol. Biol., 273, 283–298, https://doi.org/10.1006/jmbi.1997.1284, 1997.
Hamada, T., Matsunaga, S., Fujiwara, M., Fujita, K., Hirota, H., Schmucki, R., Guntert, P., and Fusetani, N.: Solution structure of polytheonamide B, a highly cytotoxic nonribosomal polypeptide from marine sponge, J. Am. Chem. Soc., 132, 12941–12945, https://doi.org/10.1021/ja104616z, 2010.
Hoesl, M. G. and Budisa, N.: In vivo incorporation of multiple noncanonical amino acids into proteins, Angew. Chem. Int. Edit., 50, 2896–2902, https://doi.org/10.1002/anie.201005680, 2011.
Hoyt, E. A., Cal, P. M. S. D., Oliveira, B. L., and Bernardes, G. J. L.: Contemporary approaches to site-selective protein modification, Nature Reviews Chemistry, 3, 147–171, https://doi.org/10.1038/s41570-019-0079-1, 2019.
Huang, Y., Russell, W. K., Wan, W., Pai, P. J., Russell, D. H., and Liu, W.: A convenient method for genetic incorporation of multiple noncanonical amino acids into one protein in Escherichia coli, Mol. Biosyst., 6, 683–686, https://doi.org/10.1039/b920120c, 2010.
IUPAC, Division of chemical nomenclature and structure representation: Specification of Configuration and Conformation, in: Nomenclature of Organic Chemistry, edited by: Favre, H. A. and Powell, W. H., The Royal Society of Chemistry, https://doi.org/10.1039/9781849733069-01156, 2013.
IUPAC-IUB Joint Commission on Biochemical Nomenclature (JCBN): Nomenclature and symbolism for amino acids and peptides. Recommendations 1983, Biochem. J., 219, 345–373, https://doi.org/10.1042/bj2190345, 1984.
Johnson, J. A., Lu, Y. Y., Van Deventer, J. A., and Tirrell, D. A.: Residue-specific incorporation of non-canonical amino acids into proteins: recent developments and applications, Curr. Opin. Chem. Biol., 14, 774–780, https://doi.org/10.1016/j.cbpa.2010.09.013, 2010.
Koziara, K. B., Stroet, M., Malde, A. K., and Mark, A. E.: Testing and validation of the Automated Topology Builder (ATB) version 2.0: prediction of hydration free enthalpies, J. Comput. Aid. Mol. Des., 28, 221–233, https://doi.org/10.1007/s10822-014-9713-7, 2014.
Kuschert, S. and Stroet, M.: ATB-UQ/CYANA-Examples: v1.0.1 (v1.0.1), Zenodo [code], https://doi.org/10.5281/zenodo.7655279, 2023.
Lee, W., Rahimi, M., Lee, Y., and Chiu, A.: POKY: a software suite for multidimensional NMR and 3D structure calculation of biomolecules, Bioinformatics, 37, 3041–3042, https://doi.org/10.1093/bioinformatics/btab180, 2021.
Link, A. J., Mock, M. L., and Tirrell, D. A.: Non-canonical amino acids in protein engineering, Curr. Opin. Biotech., 14, 603–609, https://doi.org/10.1016/j.copbio.2003.10.011, 2003.
Liu, C. C. and Schultz, P. G.: Adding new chemistries to the genetic code, Annu. Rev. Biochem., 79, 413–444, https://doi.org/10.1146/annurev.biochem.052308.105824, 2010.
Loll, P. J., Upton, E. C., Nahoum, V., Economou, N. J., and Cocklin, S.: The high resolution structure of tyrocidine A reveals an amphipathic dimer, Biochim. Biophys. Acta, 1838, 1199–1207, https://doi.org/10.1016/j.bbamem.2014.01.033, 2014.
Mal, T. K., Bagby, S., and Ikura, M.: Protein structure calculation from NMR data, Methods Mol. Biol., 173, 267–283, https://doi.org/10.1385/1-59259-184-1:267, 2002.
Malde, A. K., Zuo, L., Breeze, M., Stroet, M., Poger, D., Nair, P. C., Oostenbrink, C., and Mark, A. E.: An Automated Force Field Topology Builder (ATB) and Repository: Version 1.0, J. Chem. Theory Comput., 7, 4026–4037, https://doi.org/10.1021/ct200196m, 2011.
Marahiel, M. A.: Working outside the protein-synthesis rules: insights into non-ribosomal peptide synthesis, J. Pept. Sci., 15, 799–807, https://doi.org/10.1002/psc.1183, 2009.
Mareuil, F., Malliavin, T. E., Nilges, M., and Bardiaux, B.: Improved reliability, accuracy and quality in automated NMR structure calculation with ARIA, J. Biomol. NMR, 62, 425–438, https://doi.org/10.1007/s10858-015-9928-5, 2015.
Markley, J. L., Bax, A., Arata, Y., Hilbers, C. W., Kaptein, R., Sykes, B. D., Wright, P. E., and Wuthrich, K.: Recommendations for the presentation of NMR structures of proteins and nucleic acids. IUPAC-IUBMB-IUPAB Inter-Union Task Group on the Standardization of Data Bases of Protein and Nucleic Acid Structures Determined by NMR Spectroscopy, J. Biomol. NMR, 12, 1–23, https://doi.org/10.1023/a:1008290618449, 1998.
Martínez-Núñez, M. A. and López, V. E. L. y.: Nonribosomal peptides synthetases and their applications in industry, Sustainable Chemical Processes, 4, 13, https://doi.org/10.1186/s40508-016-0057-6, 2016.
Mekkattu Tharayil, S., Mahawaththa, M. C., Feintuch, A., Maleckis, A., Ullrich, S., Morewood, R., Maxwell, M. J., Huber, T., Nitsche, C., Goldfarb, D., and Otting, G.: Site-selective generation of lanthanoid binding sites on proteins using 4-fluoro-2,6-dicyanopyridine, Magn. Reson., 3, 169–182, https://doi.org/10.5194/mr-3-169-2022, 2022.
Mendive-Tapia, L., Preciado, S., Garcia, J., Ramon, R., Kielland, N., Albericio, F., and Lavilla, R.: New peptide architectures through C-H activation stapling between tryptophan-phenylalanine/tyrosine residues, Nat. Commun., 6, 7160, https://doi.org/10.1038/ncomms8160, 2015.
Mobli, M. and Stroet, M.: ATB-UQ/CYSX: v1.0, Zenodo [code], https://doi.org/10.5281/zenodo.7649886, 2023.
Mobli, M., Kuschert, S., and Stroet, M.: ATB-UQ/fixnom: v1.0.1, Zenodo [code], https://doi.org/10.5281/zenodo.7655274, 2023.
Noren, C. J., Anthonycahill, S. J., Griffith, M. C., and Schultz, P. G.: A General-Method for Site-Specific Incorporation of Unnatural Amino-Acids into Proteins, Science, 244, 182–188, https://doi.org/10.1126/science.2649980, 1989.
Ramanujam, V., Shen, Y., Ying, J., and Mobli, M.: Residual Dipolar Couplings for Resolving Cysteine Bridges in Disulfide-Rich Peptides, Front. Chem., 7, 889, https://doi.org/10.3389/fchem.2019.00889, 2019.
Rosengren, J. and Stroet, M.: ATB-UQ/APP-RCM-CNS-Files: v1.0, Zenodo [code], https://doi.org/10.5281/zenodo.7649851, 2023.
Schafer, M., Schneider, T. R., and Sheldrick, G. M.: Crystal structure of vancomycin, Structure, 4, 1509–1515, https://doi.org/10.1016/s0969-2126(96)00156-6, 1996.
Schmid, N., Christ, C. D., Christen, M., Eichenberger, A. P., and van Gunsteren, W. F.: Architecture, implementation and parallelisation of the GROMOS software for biomolecular simulation, Comput. Phys. Commun., 183, 890–903, https://doi.org/10.1016/j.cpc.2011.12.014, 2012.
Schwieters, C. D., Kuszewski, J. J., Tjandra, N., and Clore, G. M.: The Xplor-NIH NMR molecular structure determination package, J. Magn. Reson., 160, 65–73, https://doi.org/10.1016/s1090-7807(02)00014-9, 2003.
Schwieters, C. D., Kuszewski, J. J., and Clore, G. M.: Using Xplor-NIH for NMR molecular structure determination, Prog. Nucl. Mag. Res. Sp., 48, 47–62, https://doi.org/10.1016/j.pnmrs.2005.10.001, 2006.
Shen, Y. and Bax, A.: Protein backbone and sidechain torsion angles predicted from NMR chemical shifts using artificial neural networks, J. Biomolecular NMR, 56, 227–241, https://doi.org/10.1007/s10858-013-9741-y, 2013.
Skinner, S. P., Fogh, R. H., Boucher, W., Ragan, T. J., Mureddu, L. G., and Vuister, G. W.: CcpNmr AnalysisAssign: a flexible platform for integrated NMR analysis, J. Biomol. NMR, 66, 111–124, https://doi.org/10.1007/s10858-016-0060-y, 2016.
Strieker, M., Tanovic, A., and Marahiel, M. A.: Nonribosomal peptide synthetases: structures and dynamics, Curr. Opin. Struc. Biol., 20, 234–240, https://doi.org/10.1016/j.sbi.2010.01.009, 2010.
Stroet, M., Caron, B., Visscher, K. M., Geerke, D. P., Malde, A. K., and Mark, A. E.: Automated Topology Builder Version 3.0: Prediction of Solvation Free Enthalpies in Water and Hexane, J. Chem. Theory Comput., 14, 5834–5845, https://doi.org/10.1021/acs.jctc.8b00768, 2018.
Tharp, J. M., Krahn, N., Varshney, U., and Soll, D.: Hijacking Translation Initiation for Synthetic Biology, Chembiochem, 21, 1387–1396, https://doi.org/10.1002/cbic.202000017, 2020.
Thompson, R. E. and Muir, T. W.: Chemoenzymatic Semisynthesis of Proteins, Chem. Rev., 120, 3051–3126, https://doi.org/10.1021/acs.chemrev.9b00450, 2020.
Umstatter, F., Domhan, C., Hertlein, T., Ohlsen, K., Muhlberg, E., Kleist, C., Zimmermann, S., Beijer, B., Klika, K. D., Haberkorn, U., Mier, W., and Uhl, P.: Vancomycin Resistance Is Overcome by Conjugation of Polycationic Peptides, Angew. Chem. Int. Edit., 59, 8823–8827, https://doi.org/10.1002/anie.202002727, 2020.
Van Der Spoel, D., Lindahl, E., Hess, B., Groenhof, G., Mark, A. E., and Berendsen, H. J.: GROMACS: fast, flexible, and free, J. Comput. Chem., 26, 1701–1718, https://doi.org/10.1002/jcc.20291, 2005.
Vranken, W. F., Boucher, W., Stevens, T. J., Fogh, R. H., Pajon, A., Llinas, M., Ulrich, E. L., Markley, J. L., Ionides, J., and Laue, E. D.: The CCPN data model for NMR spectroscopy: development of a software pipeline, Proteins, 59, 687–696, https://doi.org/10.1002/prot.20449, 2005.
Walsh, C. T., Garneau-Tsodikova, S., and Gatto Jr., G. J.: Protein posttranslational modifications: the chemistry of proteome diversifications, Angew. Chem. Int. Edit., 44, 7342–7372, https://doi.org/10.1002/anie.200501023, 2005.
Wang, J., Wang, W., Kollman, P. A., and Case, D. A.: Automatic atom type and bond type perception in molecular mechanical calculations, J. Mol. Graph. Model., 25, 247–260, https://doi.org/10.1016/j.jmgm.2005.12.005, 2006.
Wang, L., Brock, A., Herberich, B., and Schultz, P. G.: Expanding the genetic code of Escherichia coli, Science, 292, 498–500, https://doi.org/10.1126/science.1060077, 2001.
Wang, T., Liang, C., An, Y., Xiao, S., Xu, H., Zheng, M., Liu, L., Wang, G., and Nie, L.: Engineering the Translational Machinery for Biotechnology Applications, Mol. Biotechnol., 62, 219–227, https://doi.org/10.1007/s12033-020-00246-y, 2020.
Weber, C., Wider, G., von Freyberg, B., Traber, R., Braun, W., Widmer, H., and Wuthrich, K.: The NMR structure of cyclosporin A bound to cyclophilin in aqueous solution, Biochemistry, 30, 6563–6574, https://doi.org/10.1021/bi00240a029, 1991.
Westbrook, J. D., Shao, C., Feng, Z., Zhuravleva, M., Velankar, S., and Young, J.: The chemical component dictionary: complete descriptions of constituent molecules in experimentally determined 3D macromolecules in the Protein Data Bank, Bioinformatics, 31, 1274–1278, https://doi.org/10.1093/bioinformatics/btu789, 2015.
Wu, Y., Li, Y. H., Li, X., Zou, Y., Liao, H. L., Liu, L., Chen, Y. G., Bierer, D., and Hu, H. G.: A novel peptide stapling strategy enables the retention of ring-closing amino acid side chains for the Wnt/beta-catenin signalling pathway, Chem. Sci., 8, 7368–7373, https://doi.org/10.1039/c7sc02420g, 2017.
Yilmaz, E. M. and Guntert, P.: NMR structure calculation for all small molecule ligands and non-standard residues from the PDB Chemical Component Dictionary, J. Biomol. NMR, 63, 21–37, https://doi.org/10.1007/s10858-015-9959-y, 2015.
Zou, H., Li, L., Zhang, T., Shi, M., Zhang, N., Huang, J., and Xian, M.: Biosynthesis and biotechnological application of non-canonical amino acids: Complex and unclear, Biotechnol. Adv., 36, 1917–1927, https://doi.org/10.1016/j.biotechadv.2018.07.008, 2018.